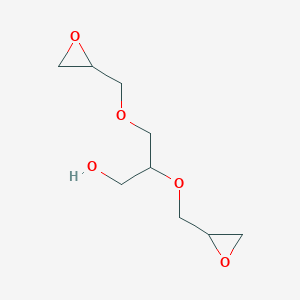
Glycerol diglycidyl ether
描述
Glycerol diglycidyl ether (GDE) is a chemical compound with the molecular formula C9H16O5, commonly used in the production of epoxy resins. It is an aliphatic epoxy monomer that serves as a diepoxy crosslinker, making it valuable in various applications, including coatings, adhesives, and composite materials. GDE is characterized by its low viscosity, which allows for easy processing and application. The compound is derived from glycerol, a naturally occurring substance, and is known for its ability to enhance the mechanical properties of cured epoxy systems.
准备方法
Reaction of Glycerol with Epichlorohydrin
This method involves:
Catalyst selection: Various catalysts can be used, including Lewis acids like boron trifluoride or tin(IV) tetrachloride[“].
Temperature and pressure considerations: Reaction conditions are typically controlled to optimize yield and product quality.
Reaction time optimization: The duration is adjusted based on the desired conversion rate to chlorohydrin ether.
Dehydrohalogenation of Halohydrin Ether
This step includes:
Use of alkali metal hydroxides: Typically, sodium or potassium hydroxide is employed.
Temperature control: The process is usually carried out between 30° to 60° C[“].
Solvent selection: An inert organic solvent may be used if the reaction mixture's viscosity is too high.
Catalysts in Glycerol Diglycidyl Ether Synthesis
Various catalysts play crucial roles in this compound synthesis:
Lewis acid catalysts: Boron trifluoride and tin(IV) tetrachloride are commonly used.
Phase transfer catalysts: These facilitate reactions between substances in different phases[“].
Other effective catalysts: Including divalent tin chloride for specific applications[“].
Reaction Mechanisms
The synthesis of this compound involves two main reaction mechanisms:
Formation of halohydrin ether intermediate: This occurs through the reaction of glycerol with epichlorohydrin.
Epoxide ring closure: The final step in forming the glycidyl ether structure[“].
Purification and Isolation
After synthesis, the following techniques are employed to obtain pure this compound:
Extraction techniques: To separate the product from reaction mixtures.
Distillation methods: For further purification and removal of volatile components.
Crystallization processes: Used when applicable for solid or semi-solid products.
Quality Control and Analysis
Ensuring the quality of synthesized this compound involves:
Purity assessment techniques: Such as gas chromatography or HPLC[“].
Structural confirmation methods: Including spectroscopic techniques.
Impurity identification: To ensure the product meets required specifications.
化学反应分析
Chemical Structure and Reactivity
Glycerol diglycidyl ether, with the molecular formula C₉H₁₆O₅, is characterized by its epoxy functionality. The compound's reactivity is primarily due to its epoxide rings, which are highly susceptible to nucleophilic attack. Factors influencing this compound's reactivity include temperature, pH, and the presence of catalysts.
Epoxide Ring Opening Reactions
The epoxide rings in this compound undergo nucleophilic addition reactions, leading to ring opening. This process is often stereospecific, resulting in the formation of new hydroxyl groups. The reaction can be catalyzed by both acids and bases, with the mechanism varying depending on the catalyst used[“].
Esterification Reactions
This compound can participate in esterification reactions, particularly with anhydrides. These reactions lead to the formation of internal ester groups, altering the compound's properties. The kinetics of esterification reactions involving this compound are influenced by factors such as temperature and catalyst concentration[“].
Polymerization Reactions
One of the most significant reactions of this compound is its ability to undergo polymerization. Copolymerization with aromatic dithiols is a well-studied example, resulting in the formation of crosslinked networks. The crosslinking mechanisms and the resulting polymer properties are highly dependent on reaction conditions such as temperature and stoichiometry.
Spectroscopic Analysis of Reactions
Fourier Transform Infrared (FTIR) spectroscopy is commonly used to monitor this compound reactions, providing real-time information on functional group changes. Nuclear Magnetic Resonance (NMR) spectroscopy offers detailed structural information about reaction products, while mass spectrometry aids in product identification and characterization[“].
Thermal Analysis of Reaction Products
Differential Scanning Calorimetry (DSC) is employed to study the curing behavior of this compound-based systems, providing insights into reaction kinetics and thermodynamics. Thermogravimetric Analysis (TGA) complements DSC by offering information on the thermal stability and decomposition of reaction products[“].
Reaction Kinetics and Mechanisms
The kinetics of this compound reactions are often complex, with many systems exhibiting autocatalytic behavior. Activation energy calculations and reaction order determinations are crucial for understanding these mechanisms. Advanced kinetic modeling techniques are frequently employed to elucidate the intricacies of this compound reaction pathways[“].
Catalytic Effects in this compound Reactions
Lewis acid catalysts, such as boron trifluoride, play a significant role in many this compound reactions, particularly in polymerization processes. Phase transfer catalysts can also be employed to facilitate reactions in heterogeneous systems. The concentration and type of catalyst can dramatically influence reaction rates and product distributions[“].
Environmental Considerations in Reactions
Green chemistry approaches are increasingly being applied to this compound reactions. Solvent-free reaction systems and the use of renewable catalysts are areas of active research. The biodegradability of reaction products is also a key consideration in the environmental impact assessment of this compound-based materials.
科学研究应用
Polymer Science Applications
Epoxy Resin Formulations
Glycerol diglycidyl ether plays a crucial role in epoxy resin formulations, often used as a reactive diluent. Its incorporation helps enhance specific properties of the resins, such as flexibility and adhesion promotion. These formulations find utility in a wide range of applications, including surface-coating resins, dipping resins, and impregnating resins.
Reactive Diluent in Polymer Systems
The use of this compound as a reactive diluent helps maintain lower viscosity in polymer systems while contributing to the overall properties of the final product. This characteristic is particularly valuable in applications requiring precise control over material flow and processing.
Crosslinking Agent for Thermosetting Polymers
This compound serves as an effective crosslinking agent in thermosetting polymer systems. Its ability to form chemical bonds between polymer chains contributes to the development of materials with enhanced mechanical and thermal properties[“].
Biomaterials Research
Biodegradable Polymer Development
This compound has shown promise in the development of biodegradable materials. Its use in polymer synthesis allows for the creation of materials with controlled degradation profiles, which is crucial for various biomedical applications[“].
Tissue Engineering Scaffolds
In tissue engineering, this compound-based materials have been explored for creating scaffolds that support cell growth and tissue regeneration. The ability to tailor the properties of these scaffolds makes them valuable in regenerative medicine research.
Drug Delivery Systems
The incorporation of this compound in polymer systems has been investigated for drug delivery applications. Its unique chemistry allows for the development of controlled release systems, potentially improving the efficacy of pharmaceutical treatments[“].
Surface Modification and Coatings
Adhesion Promoters
This compound's epoxy functionality makes it an excellent candidate for use as an adhesion promoter in various coating applications. This property enhances the bonding between different materials, improving the overall performance of composite structures.
Corrosion-Resistant Coatings
Research has explored the use of this compound in developing corrosion-resistant coatings. Its ability to form crosslinked networks contributes to the creation of protective barriers against environmental degradation.
Functionalization of Nanoparticles
This compound has been utilized in the functionalization of nanoparticles, allowing for the modification of surface properties and the creation of novel nanocomposite materials[“].
Environmental Science Applications
Eco-Friendly Alternatives to Petroleum-Based Chemicals
As a bio-based compound, this compound represents an eco-friendly alternative to petroleum-derived chemicals in various applications. This aligns with the growing trend towards sustainable and renewable resources in chemical production[“].
Valorization of Biorefinery By-Products
This compound synthesis from glycerol, a by-product of biodiesel production, contributes to the valorization of biorefinery waste streams. This approach enhances the overall efficiency and sustainability of biofuel production processes[“].
作用机制
Chemical Structure and Reactivity
Glycerol diglycidyl ether's molecular structure consists of a glycerol backbone with two epoxide rings attached. These epoxide groups are highly reactive due to the strain in their three-membered rings. The reactivity of this compound is influenced by factors such as temperature, pH, and the presence of catalysts[“].
Epoxide Ring Opening Mechanism
The primary mechanism of action for this compound involves the opening of its epoxide rings. This process typically occurs through nucleophilic addition, where a nucleophile attacks the less substituted carbon of the epoxide ring. The stereochemistry of this ring opening is often influenced by the nature of the nucleophile and reaction conditions.
Catalysts, particularly Lewis acids, can significantly affect the ring opening mechanism by coordinating with the epoxide oxygen and increasing its electrophilicity[“].
Crosslinking Mechanism
In polymer systems, this compound acts as a crosslinking agent. The epoxy groups of this compound react with functional groups on polymer chains, forming chemical bonds that link different chains together. This crosslinking mechanism is crucial in the formation of thermoset polymers and is influenced by temperature and the presence of catalysts[“].
Esterification Mechanism
This compound can undergo esterification reactions, particularly with anhydrides, leading to the formation of internal ester groups. This mechanism involves the nucleophilic attack of the epoxide ring by the anhydride, followed by ring opening and ester formation. The kinetics of this process depend on factors such as temperature and catalyst concentration[“].
Polymerization Mechanism
In polymerization reactions, this compound can act as both a monomer and a crosslinking agent. The polymerization mechanism typically involves three stages: initiation, propagation, and termination. The epoxy groups of this compound participate in chain growth reactions, leading to the formation of complex polymer networks[“].
Mechanism in Nanocomposite Formation
This compound plays a crucial role in the formation of nanocomposites by facilitating interfacial bonding between the polymer matrix and nanoparticles. The epoxy groups can react with surface functionalities on nanoparticles, creating strong covalent bonds that enhance the properties of the resulting nanocomposite[“].
Photocross-linking Mechanism
In the presence of photoinitiators, this compound can undergo light-induced crosslinking reactions. This mechanism involves the formation of free radicals upon light exposure, which then initiate the polymerization and crosslinking of this compound molecules. The resulting network formation is crucial in applications such as photoresists and UV-curable coatings[“].
Mechanism in Biodegradable Materials
The ester bonds formed during this compound reactions can undergo hydrolysis in aqueous environments, contributing to the biodegradability of this compound-based materials. Additionally, certain enzymes can catalyze the degradation of these materials, with the rate of biodegradation influenced by factors such as pH, temperature, and the presence of specific microorganisms.
Mechanism in Antibacterial Action
Some this compound-based materials exhibit antibacterial properties, with the mechanism involving interactions between the epoxy groups and bacterial cell walls. This interaction can lead to the disruption of cellular processes, ultimately resulting in bacterial cell death. The efficacy of this antibacterial action depends on factors such as the concentration of this compound and the type of bacteria involved[“].
相似化合物的比较
Structural Similarities and Differences
Comparison with Other Diglycidyl Ethers
Glycerol diglycidyl ether contains two epoxide groups, which are responsible for its reactivity. In comparison, other diglycidyl ethers, such as bisphenol A diglycidyl ether (BADGE), also have two epoxide groups but differ in their backbone structures. BADGE, derived from bisphenol A, has a more rigid structure compared to the flexible glycerol backbone of this compound, which can influence the properties of the resulting materials.
Comparison with Monoglycidyl Ethers
Monoglycidyl ethers contain only one epoxide group, making them less reactive than diglycidyl ethers like this compound. This structural difference results in distinct applications; monoglycidyl ethers are often used as reactive diluents in epoxy formulations, whereas this compound's two epoxide groups enable it to act as a crosslinking agent.
Comparison with Triglycidyl Ethers
Triglycidyl ethers contain three epoxide groups, providing higher functionality than this compound. This increased functionality can lead to denser crosslinking in polymer networks. However, the higher viscosity of triglycidyl ethers may limit their use in certain applications compared to the more manageable viscosity of this compound.
Reactivity Comparison
Epoxide Ring Reactivity
The reactivity of the epoxide ring is a critical factor in determining how these compounds behave in chemical reactions. This compound's epoxide rings are highly reactive due to the strain inherent in their three-membered structure. In comparison, other diglycidyl ethers like BADGE may exhibit slightly different reactivity profiles due to their structural differences.
Crosslinking Capabilities
This compound is known for its excellent crosslinking capabilities, making it suitable for creating thermosetting polymers. Compared to monoglycidyl ethers, which have limited crosslinking potential due to their single epoxide group, this compound provides enhanced mechanical properties in cured materials. Triglycidyl ethers offer even greater crosslinking density but may result in increased brittleness.
Comparison of Reaction Rates
The reaction rates of this compound can vary depending on the presence of catalysts and reaction conditions. Studies indicate that this compound reacts more rapidly than some other glycidyl ethers under similar conditions due to its dual epoxide functionality, which facilitates faster curing processes.
Comparison with Bisphenol A Diglycidyl Ether
Structural Differences
This compound differs structurally from bisphenol A diglycidyl ether (BADGE) primarily in its backbone composition. While BADGE is derived from bisphenol A and exhibits a rigid structure, this compound features a glycerol backbone that allows for greater flexibility and adaptability in various formulations.
Toxicity Comparison
Toxicity is an important consideration when evaluating chemical compounds. Research has shown that BADGE can release bisphenol A during degradation, raising concerns about its safety. In contrast, glycerol-based compounds like this compound are generally considered safer alternatives due to their lower toxicity profiles and reduced environmental impact.
Environmental Impact Comparison
The environmental impact of these compounds also varies significantly. This compound, being derived from renewable resources like glycerol, presents a more sustainable option compared to BADGE, which is derived from petroleum-based sources. This aspect makes this compound a favorable choice in green chemistry initiatives.
Comparison with Ethylene Glycol Diglycidyl Ether
Molecular Weight Differences
Ethylene glycol diglycidyl ether (EGDGE) has a lower molecular weight compared to this compound, which influences its viscosity and handling characteristics. The lower viscosity of EGDGE allows for easier processing but may result in less effective crosslinking compared to the higher molecular weight of this compound.
Viscosity Comparison
The viscosity of a compound plays a crucial role in its application. This compound typically exhibits higher viscosity than EGDGE due to its larger molecular size and structure. This difference can affect how each compound is used in formulations; for example, EGDGE may be preferred for applications requiring low-viscosity materials.
Application Versatility
Both this compound and EGDGE are versatile compounds used in various applications; however, this compound's unique properties allow it to serve effectively as both a reactive diluent and a crosslinking agent, whereas EGDGE is often limited to specific roles within epoxy systems.
Comparison with Propylene Glycol Diglycidyl Ether
Structural Similarities
Propylene glycol diglycidyl ether (PGDGE) shares structural similarities with this compound as both compounds contain two epoxide groups. However, PGDGE is derived from propylene glycol rather than glycerol, leading to differences in their physical properties and reactivity profiles.
常见问题
How does glycerol diglycidyl ether react with amines?
This compound reacts with amines through a nucleophilic addition mechanism, where the amine attacks the epoxide ring, leading to ring opening and the formation of a β-hydroxy amine.
What is the mechanism of curing in epoxy resins containing this compound?
The curing mechanism involves the crosslinking of epoxy groups with hardeners, typically amines or anhydrides, resulting in a three-dimensional network structure.
How does the mechanism of action differ in aqueous and non-aqueous environments?
In aqueous environments, hydrolysis of the epoxide rings can compete with other reactions, while in non-aqueous environments, direct nucleophilic addition is more prevalent.
What role does the mechanism of this compound play in its biodegradability?
The ester bonds formed during this compound reactions can undergo hydrolysis, facilitating the breakdown of this compound-based materials in the environment.
How does the mechanism of this compound contribute to its use in nanocomposites?
This compound's epoxy groups can react with surface functionalities on nanoparticles, creating strong interfacial bonds that enhance the properties of nanocomposites.
How does this compound compare to other epoxy compounds in terms of reactivity?
This compound exhibits high reactivity due to its two epoxide groups compared to monoglycidyl ethers but may react slower than triglycidyl ethers because they have more reactive sites available.
What are the main differences between this compound and bisphenol A diglycidyl ether?
The primary differences lie in their structures; BADGE has a rigid backbone leading potentially higher toxicity levels while this compound offers flexibility and lower toxicity.
How does the biodegradability of this compound compare with other similar compounds?
This compound is generally more biodegradable than petroleum-based glycidyl ethers because it breaks down more easily into simpler substances via microbial action.
What advantages does this compound have over other diglycidyl ethers in polymer formulations?
Its advantages include better flexibility and toughness while maintaining manageable viscosity during processing.
How does the biocompatibility of this compound compare with other similar compounds?
It tends to exhibit lower cytotoxicity and allergenic potential than traditional epoxy resins like BADGE, making it suitable for biomedical applications where safety is critical.
属性
IUPAC Name |
2,3-bis(oxiran-2-ylmethoxy)propan-1-ol | |
---|---|---|
Details | Computed by LexiChem 2.6.6 (PubChem release 2019.06.18) | |
Source | PubChem | |
URL | https://pubchem.ncbi.nlm.nih.gov | |
Description | Data deposited in or computed by PubChem | |
InChI |
InChI=1S/C9H16O5/c10-1-7(12-5-9-6-14-9)2-11-3-8-4-13-8/h7-10H,1-6H2 | |
Details | Computed by InChI 1.0.5 (PubChem release 2019.06.18) | |
Source | PubChem | |
URL | https://pubchem.ncbi.nlm.nih.gov | |
Description | Data deposited in or computed by PubChem | |
InChI Key |
IVIDDMGBRCPGLJ-UHFFFAOYSA-N | |
Details | Computed by InChI 1.0.5 (PubChem release 2019.06.18) | |
Source | PubChem | |
URL | https://pubchem.ncbi.nlm.nih.gov | |
Description | Data deposited in or computed by PubChem | |
Canonical SMILES |
C1C(O1)COCC(CO)OCC2CO2 | |
Details | Computed by OEChem 2.1.5 (PubChem release 2019.06.18) | |
Source | PubChem | |
URL | https://pubchem.ncbi.nlm.nih.gov | |
Description | Data deposited in or computed by PubChem | |
Molecular Formula |
C9H16O5 | |
Details | Computed by PubChem 2.1 (PubChem release 2019.06.18) | |
Source | PubChem | |
URL | https://pubchem.ncbi.nlm.nih.gov | |
Description | Data deposited in or computed by PubChem | |
Molecular Weight |
204.22 g/mol | |
Details | Computed by PubChem 2.1 (PubChem release 2021.05.07) | |
Source | PubChem | |
URL | https://pubchem.ncbi.nlm.nih.gov | |
Description | Data deposited in or computed by PubChem | |
CAS No. |
27043-36-3 | |
Record name | Glycerol diglycidyl ether | |
Source | European Chemicals Agency (ECHA) | |
URL | https://echa.europa.eu/information-on-chemicals | |
Description | The European Chemicals Agency (ECHA) is an agency of the European Union which is the driving force among regulatory authorities in implementing the EU's groundbreaking chemicals legislation for the benefit of human health and the environment as well as for innovation and competitiveness. | |
Explanation | Use of the information, documents and data from the ECHA website is subject to the terms and conditions of this Legal Notice, and subject to other binding limitations provided for under applicable law, the information, documents and data made available on the ECHA website may be reproduced, distributed and/or used, totally or in part, for non-commercial purposes provided that ECHA is acknowledged as the source: "Source: European Chemicals Agency, http://echa.europa.eu/". Such acknowledgement must be included in each copy of the material. ECHA permits and encourages organisations and individuals to create links to the ECHA website under the following cumulative conditions: Links can only be made to webpages that provide a link to the Legal Notice page. | |
Retrosynthesis Analysis
AI-Powered Synthesis Planning: Our tool employs the Template_relevance Pistachio, Template_relevance Bkms_metabolic, Template_relevance Pistachio_ringbreaker, Template_relevance Reaxys, Template_relevance Reaxys_biocatalysis model, leveraging a vast database of chemical reactions to predict feasible synthetic routes.
One-Step Synthesis Focus: Specifically designed for one-step synthesis, it provides concise and direct routes for your target compounds, streamlining the synthesis process.
Accurate Predictions: Utilizing the extensive PISTACHIO, BKMS_METABOLIC, PISTACHIO_RINGBREAKER, REAXYS, REAXYS_BIOCATALYSIS database, our tool offers high-accuracy predictions, reflecting the latest in chemical research and data.
Strategy Settings
Precursor scoring | Relevance Heuristic |
---|---|
Min. plausibility | 0.01 |
Model | Template_relevance |
Template Set | Pistachio/Bkms_metabolic/Pistachio_ringbreaker/Reaxys/Reaxys_biocatalysis |
Top-N result to add to graph | 6 |
Feasible Synthetic Routes
体外研究产品的免责声明和信息
请注意,BenchChem 上展示的所有文章和产品信息仅供信息参考。 BenchChem 上可购买的产品专为体外研究设计,这些研究在生物体外进行。体外研究,源自拉丁语 "in glass",涉及在受控实验室环境中使用细胞或组织进行的实验。重要的是要注意,这些产品没有被归类为药物或药品,他们没有得到 FDA 的批准,用于预防、治疗或治愈任何医疗状况、疾病或疾病。我们必须强调,将这些产品以任何形式引入人类或动物的身体都是法律严格禁止的。遵守这些指南对确保研究和实验的法律和道德标准的符合性至关重要。