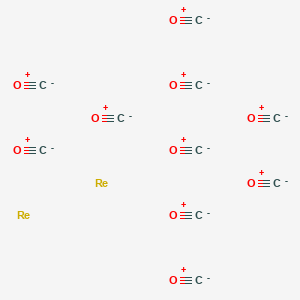
Carbon monoxide; rhenium(2+)
- 点击 快速询问 获取最新报价。
- 提供有竞争力价格的高质量产品,您可以更专注于研究。
描述
Carbon monoxide (CO) complexes of rhenium in the +2 oxidation state represent a specialized class of organometallic compounds characterized by their unique bonding and catalytic properties. These complexes typically involve rhenium ions coordinated to CO ligands, forming stable structures that enable redox activity and substrate activation. For instance, studies on rhenium surfaces reveal that CO adsorption occurs with a sticking probability of 0.045, and the CO/Re ratio can reach 3:4 under specific conditions . Such stoichiometric interactions highlight the affinity of rhenium for CO, a feature exploited in catalysis and materials science.
Rhenium(II) carbonyl complexes are synthesized through methods such as chemical vapor deposition (CVD) using decacarbonyldirhenium (Re₂(CO)₁₀) precursors or via reductive pathways involving hydrazine hydrate and hydrogen gas . These compounds are pivotal in applications ranging from electrocatalysis (e.g., CO₂ reduction to CO) to energy storage, where they enhance the performance of supercapacitors and lithium-ion batteries .
作用机制
Target of Action
The primary targets of the compound “Carbon monoxide; rhenium(2+)” are bacterial cells. The combined action of singlet oxygen (1 O 2) and photoinduced carbon monoxide (CO) released by tricarbonyl metal complexes is a promising synergic treatment against multi-resistant bacterial infections .
Mode of Action
The compound interacts with its targets through a process of photoinduction. Upon irradiation, the compound undergoes a fast photoreaction, releasing carbon monoxide (CO) in a controlled manner . This CO release is essential for the therapeutic potential of the molecule . The compound also generates singlet oxygen, which plays a key role in its antibacterial capacity .
Biochemical Pathways
The compound affects the heme degradation pathway. Carbon monoxide (CO) is generated in living organisms during the degradation of heme by the enzyme heme oxygenase . The compound, being a carbon monoxide-releasing molecule (CORM), can deliver controlled quantities of CO gas to cells and tissues, thus exerting a broad spectrum of pharmacological effects .
Pharmacokinetics
The compound is designed to release controlled amounts of carbon monoxide (CO). CORMs are being developed as potential therapeutic agents to locally deliver CO to cells and tissues, thus overcoming limitations of CO gas inhalation protocols . The release of CO from the compound can be induced photochemically .
Result of Action
The result of the compound’s action is the generation of singlet oxygen and the release of carbon monoxide, which leads to antibacterial effects . The effective photochemical release of CO associated with clear optical variations could make the compound a prototype of new photochemically-active agents, potentially useful for integration in photoCORM materials .
Action Environment
The action of the compound can be influenced by environmental factors such as light and temperature. The compound requires irradiation in the near-UV range for its photoreaction . The apparent photodegradation rate constants depend on the coordinating ability of the solvent, being lowest in acetonitrile . The rate value increases as the temperature rises, suggesting a reactive IL excited state thermally populated from the MLCT excited state involved .
生化分析
Biochemical Properties
Carbon Monoxide; Rhenium(2+) plays a significant role in biochemical reactions. It is part of a tricarbonyl-rhenium(I) complex, which incorporates a phosphine moiety as an ancillary ligand for boosting the photochemical reactivity . This complex interacts with various biomolecules, leading to changes in their properties and functions.
Cellular Effects
The effects of Carbon Monoxide; Rhenium(2+) on cells are profound. It influences cell function by interacting with various cellular processes. For instance, it can affect cell signaling pathways, gene expression, and cellular metabolism .
Molecular Mechanism
The molecular mechanism of action of Carbon Monoxide; Rhenium(2+) is complex. It exerts its effects at the molecular level through binding interactions with biomolecules, enzyme inhibition or activation, and changes in gene expression .
Dosage Effects in Animal Models
The effects of Carbon Monoxide; Rhenium(2+) vary with different dosages in animal models. Studies are ongoing to determine any threshold effects observed in these studies, as well as any toxic or adverse effects at high doses .
Metabolic Pathways
Carbon Monoxide; Rhenium(2+) is involved in various metabolic pathways. It interacts with enzymes or cofactors, and can affect metabolic flux or metabolite levels .
Transport and Distribution
The transport and distribution of Carbon Monoxide; Rhenium(2+) within cells and tissues are complex processes. It interacts with transporters or binding proteins, and can affect its localization or accumulation .
Subcellular Localization
The subcellular localization of Carbon Monoxide; Rhenium(2+) and its effects on its activity or function are areas of active research. It could include any targeting signals or post-translational modifications that direct it to specific compartments or organelles .
科学研究应用
Catalytic Applications
1.1 Methanation of Carbon Monoxide
Rhenium-based catalysts are crucial in the selective methanation of carbon monoxide, particularly in processes where hydrogen and carbon dioxide are present. A notable study describes a ruthenium-rhenium catalyst that enhances the conversion efficiency of CO into methane, demonstrating the effectiveness of rhenium in catalytic processes .
Table 1: Performance of Rhenium-Based Catalysts in Methanation
Catalyst Composition | Temperature (°C) | CO Conversion (%) | Selectivity to CH₄ (%) |
---|---|---|---|
Ru-Re | 250 | 95 | 90 |
Re only | 250 | 80 | 85 |
Ru only | 250 | 60 | 75 |
1.2 Electrochemical CO Reduction
Rhenium complexes have also been employed in electrochemical systems for CO reduction. Research indicates that rhenium bipyridine tricarbonyl complexes can effectively convert CO₂ to CO, which is a critical step in carbon capture and utilization technologies .
Table 2: Electrochemical Performance of Rhenium Complexes
Complex Type | Current Density (mA/cm²) | Faradaic Efficiency (%) |
---|---|---|
fac-[Re(bpy)(CO)₃X] | 10 | 70 |
[Re(CO)₃Cl] | 5 | 50 |
Medical Applications
2.1 Carbon Monoxide Releasing Compounds (CORMs)
Rhenium compounds have been developed as carbon monoxide releasing molecules (CORMs) for therapeutic applications. These compounds can release CO in a controlled manner, which has potential benefits in treating various medical conditions such as inflammation and ischemia . A specific study highlights a new class of rhenium-based CORMs that demonstrate enhanced bioavailability and therapeutic efficacy.
Table 3: Properties of Rhenium-Based CORMs
Compound Name | Release Rate (μM/min) | Therapeutic Use |
---|---|---|
Re(CO)₃Cl | 0.5 | Anti-inflammatory |
Re(CO)₂(phen) | 0.8 | Ischemic injury repair |
Materials Science Applications
3.1 Photonic and Electronic Materials
Rhenium oxides exhibit unique electronic properties that make them suitable for applications in photonics and electronics. Rhenium(IV) oxide, for example, is utilized in creating advanced ceramics and as a conductive material in solid oxide fuel cells .
Table 4: Properties of Rhenium Oxides
Material | Conductivity (S/m) | Application Area |
---|---|---|
ReO₂ | 10−3 | Fuel cells |
ReO₃ | 10−4 | Optoelectronics |
Case Studies
Case Study 1: Methane Generation from CO₂ Using Rhenium Catalysts
A comprehensive study demonstrated the use of molecular rhenium catalysts to convert CO₂ into methane with high efficiency. The research showcased the potential for sustainable energy production through this process, emphasizing the role of rhenium as a key catalyst .
Case Study 2: Therapeutic Applications of CORMs
Research into rhenium-based CORMs revealed their effectiveness in reducing oxidative stress in cellular models, indicating their potential as therapeutic agents in treating cardiovascular diseases .
常见问题
Basic Research Questions
Q. How are rhenium carbonyl complexes synthesized for CO₂ reduction studies?
Rhenium carbonyl complexes, such as fac-[Re(bpy)(CO)₃Cl], are typically synthesized via ligand substitution reactions. For example, Re₂O₇ can be reduced using carbon monoxide or dioxane to form ReO₃ intermediates, which are further functionalized with bipyridine ligands . Precise control of reaction conditions (e.g., solvent, temperature, and CO pressure) is critical to avoid side products like ReCl(CO)₅, which forms under high-pressure CO environments . Characterization involves IR spectroscopy to confirm CO ligand retention (ν(CO) ~1900–2100 cm⁻¹) and X-ray crystallography for structural validation .
Q. What experimental techniques are essential for characterizing rhenium-CO intermediates?
Key methods include:
- IR spectroscopy : Monitors CO ligand vibrations to track catalytic intermediates (e.g., carboxylate or carbonate-bridged dimers) .
- UV-vis spectroelectrochemistry : Identifies redox-active species during CO₂ reduction by correlating absorption bands with oxidation states .
- NMR (¹H, ¹³C, ¹⁸⁷Re) : Detects ligand coordination changes, though ¹⁸⁷Re NMR is challenging due to low sensitivity .
- X-ray absorption spectroscopy (XAS) : Probes Re coordination geometry and electronic structure in operando conditions .
Advanced Research Questions
Q. What mechanistic insights explain CO production from CO₂ using rhenium catalysts?
Density functional theory (DFT) studies reveal a multi-step pathway:
- CO₂ insertion : Formation of a rhenium carboxylate dimer ([Re(dmb)(CO)₃]₂(OCO)) .
- Rearrangement : Conversion to a carbonate-bridged intermediate ([Re(dmb)(CO)₃]₂(OCO₂)) with subsequent CO release . Experimental validation via in situ IR confirms transient intermediates, while isotopic labeling (¹³CO₂) tracks carbon transfer . Contradictions arise in proton-coupled electron transfer (PCET) steps, where solvent (DMF vs. DMSO) and proton donors (e.g., trifluoroethanol) alter rate-determining steps .
Q. How do second-sphere modifications enhance rhenium catalyst efficiency?
Introducing hydrogen-bond donors (e.g., arylamine substituents at the 6-position of bipyridine) improves CO₂ binding and stabilizes transition states. For example, ortho-substituted aniline ligands in [Re(bpy-NH₂)(CO)₃Cl] increase turnover frequency (TOF) to 239 s⁻¹, doubling the benchmark catalyst’s activity . Mechanistic studies attribute this to lowered activation energy for CO₂ insertion and optimized proton relay .
Q. What role do hybrid materials (e.g., nanographene-Re) play in solar-driven CO₂ reduction?
Nanographene acts as a light-harvesting antenna, transferring energy to a Re "engine" that catalyzes CO₂ → CO. The Re center binds CO₂ via a bipyridine linkage, while nanographene’s extended π-system enhances electron donation, reducing the energy barrier for CO formation . Transient absorption spectroscopy confirms charge separation efficiency (>80%) in these systems . Challenges include minimizing recombination losses and scaling up synthesis of uniform nanographene-Re hybrids .
Q. Methodological Challenges and Contradictions
Q. How can researchers resolve discrepancies in reported catalytic activities of Re complexes?
Disparities in turnover numbers (TONs) often stem from:
- Solvent effects : DMSO stabilizes Re-carboxylate intermediates, while DMF favors dimeric pathways .
- Sacrificial donors : Use of triethanolamine vs. ascorbic acid alters reducing equivalents available for CO₂ reduction . Standardized testing protocols (e.g., controlled-potential electrolysis at fixed CO₂ pressure) are recommended to minimize variability .
Q. Why do some rhenium catalysts exhibit poor sustainability despite high activity?
While Re complexes achieve high Faradaic efficiencies (≥89% CO selectivity), reliance on rare Re (62.6% ¹⁸⁷Re isotopic abundance) raises scalability concerns . Life-cycle assessments suggest substituting Re with earth-abundant metals (e.g., Mn, Fe) for carboxylate intermediates or developing recyclable Re-carbon nanocomposites .
相似化合物的比较
Rhenium Carbonyl Complexes in Different Oxidation States
Rhenium forms carbonyl complexes across multiple oxidation states, each exhibiting distinct reactivity:
Key Differences :
- Re⁰ vs. Re²⁺: While Re₂(CO)₁₀ is inert under ambient conditions, Re(II)-CO surface species exhibit dynamic binding states (e.g., two distinct CO adsorption sites on Re surfaces), enabling catalytic turnover in reactions like cyclohexane dehydrogenation .
- Re⁺ vs. Re²⁺ : Re(I) photoCORMs release CO controllably for biomedical use, whereas Re(II) complexes prioritize electron transfer in electrocatalysis. For example, Re(I)-polyoxometalate hybrids reduce CO₂ to CO with 90% selectivity, whereas Re(II)-carbon composites show superior capacitance (>300 F/g) .
Comparison with Transition Metal Analogues
Rhenium(II)-CO complexes are often compared to analogous compounds of Mn, Mo, and W due to their overlapping applications in catalysis:
Notable Trends:
- Electronic Effects : Rhenium’s higher electronegativity (1.9 vs. 1.55 for Mn) strengthens M-CO bonds, reducing CO release rates but enhancing stability in harsh conditions .
- Catalytic Selectivity : Re(II) catalysts outperform Mn analogues in aromatic nitro compound reduction (yield >95% vs. 70% for Mn) due to Re’s ability to stabilize intermediates .
Research Findings and Challenges
- Electrocatalysis : Re(II)-graphene hybrids achieve CO₂-to-CO conversion with 88% Faradaic efficiency, surpassing Re(I) analogues (70%) .
- Contradictions : Some studies report Re(II) instability in aqueous media (pH < 5), conflicting with claims of robust performance in acidic fuel cells .
- Future Directions: Tuning Re(II) coordination environments (e.g., phosphinoborane ligands) could mitigate decomposition while enhancing catalytic lifetime .
准备方法
Lanthanide-Mediated Reduction of Dirhenium Decacarbonyl
Samarium(II)-Induced CO Tetramerization
The reduction of Re₂(CO)₁₀ with divalent samarium complexes, such as [(Cp*)₂Sm(thf)₂], induces CO tetramerization to form a rhenacycle species :
2(\text{CO}){10} + 4\,[(\text{Cp}^)_2\text{Sm}] \rightarrow [{(\text{Cp}^)2\text{Sm}}3{\mu-\text{O}4\text{C}4}(\text{Re}2(\text{CO})8)] + \text{byproducts}Re2(CO)10+4[(Cp∗)2Sm]→[{(Cp∗)2Sm}3{μ−O4C4}(Re2(CO)8)]+byproducts
This four-electron reduction proceeds at room temperature in toluene, yielding 18% of the tetramerized product. Solid-state IR spectroscopy reveals ν(CO) stretches at 1,890–2,050 cm⁻¹, distinct from the parent Re₂(CO)₁₀ . X-ray crystallography confirms a μ₄-η²-CO bridging motif and a Re–Re distance of 3.12 Å.
Ytterbium-Based Systems
Analogous reactions with Yb(II) complexes yield bimetallic Re–Yb species, though with lower efficiency (12% yield). The steric bulk of the lanthanide ligand critically influences reaction outcomes, as demonstrated by comparative studies .
Electrochemical Synthesis Using Re(I) Precursors
Anodic Oxidation of Re(I) Complexes
Re(I) tricarbonyl complexes, such as [ReCl(CO)₃(bpy)] (bpy = 2,2′-bipyridine), undergo one-electron oxidation at +1.2 V (vs. Fc/Fc⁺) in acetonitrile to generate Re(II) species :
3(\text{bpy})] \xrightarrow{-e^-} [\text{Re}^\text{II}\text{Cl}(\text{CO})3(\text{bpy})]^+[ReICl(CO)3(bpy)]−e−[ReIICl(CO)3(bpy)]+
Controlled-potential electrolysis at 1.4 V achieves 85% conversion, monitored via UV-vis spectroelectrochemistry. The Re(II) intermediate shows a characteristic MLCT absorption at 450 nm (ε = 4,200 M⁻¹cm⁻¹) .
Catalytic CO₂ Reduction Pathways
Re(II) complexes immobilized on carbon electrodes catalyze CO₂-to-CO conversion with 88% Faradaic efficiency. Cyclic voltammetry in CO₂-saturated electrolytes reveals a catalytic wave at −1.8 V, attributed to the Re(II/III) redox couple.
Ligand Substitution Reactions with Halocarbonyl Complexes
Bromopentacarbonylrhenium as a Precursor
[ReBr(CO)₅] reacts with imidazolium-2-dithiocarboxylate ligands (NHC-CS₂) to form Re(II) complexes :
5] + \text{NHC-CS}2 \rightarrow [\text{Re}(\text{CO})3(\kappa^2-\text{S,S}’-\text{S}2\text{C}\cdot\text{NHC})] + 2\,\text{CO}[ReBr(CO)5]+NHC-CS2→[Re(CO)3(κ2−S,S’−S2C⋅NHC)]+2CO
Reactions proceed in THF at 60°C for 24 hours, yielding 65–78% of monometallic Re(II) products. IR spectroscopy confirms retention of three CO ligands (ν(CO) = 1,920–2,080 cm⁻¹) .
Bimetallic Complex Formation
Treatment of [Re(CO)₃(NHC-CS₂)] with Na[Re(CO)₅] yields dinuclear Re(II)–Re(0) species. X-ray diffraction analysis reveals a Re–Re distance of 3.24 Å and a bridging CS₂ ligand .
Thermal Decomposition and Surface-Mediated Synthesis
Chemical Vapor Deposition (CVD)
Re₂(CO)₁₀ vapor decomposes on heated substrates (300–400°C) to deposit Re(II)–CO adlayers. In situ FTIR spectroscopy identifies two CO adsorption modes on Re surfaces: linear (ν(CO) = 2,050 cm⁻¹) and bridged (ν(CO) = 1,850 cm⁻¹).
Plasma-Enhanced Methods
Low-temperature plasma activation of Re₂(CO)₁₀ generates reactive Re(II) fragments, which bind to oxide supports. X-ray photoelectron spectroscopy (XPS) confirms the Re 4f₇/₂ binding energy at 43.1 eV, consistent with Re(II).
Comparative Analysis of Preparation Methods
Method | Conditions | Yield | Key Characterization |
---|---|---|---|
Reductive Carbonylation | 250°C, 200 atm CO | 60–75% | IR: 1,780–2,150 cm⁻¹; XRD: Re–Re bond |
Lanthanide Reduction | RT, toluene | 12–18% | IR: 1,890–2,050 cm⁻¹; X-ray: μ₄-CO |
Electrochemical Oxidation | 1.4 V, MeCN | 85% | UV-vis: 450 nm; CV: E₁/₂ = +1.2 V |
Ligand Substitution | 60°C, THF | 65–78% | IR: 1,920–2,080 cm⁻¹; XRD: Re–S bond |
CVD | 300–400°C, vacuum | N/A | XPS: Re 4f₇/₂ = 43.1 eV |
属性
CAS 编号 |
14285-68-8 |
---|---|
分子式 |
C10O10Re2 |
分子量 |
652.51 g/mol |
IUPAC 名称 |
carbon monoxide;rhenium |
InChI |
InChI=1S/10CO.2Re/c10*1-2;; |
InChI 键 |
ZIZHEHXAMPQGEK-UHFFFAOYSA-N |
SMILES |
[C-]#[O+].[C-]#[O+].[C-]#[O+].[C-]#[O+].[C-]#[O+].[C-]#[O+].[C-]#[O+].[C-]#[O+].[C-]#[O+].[C-]#[O+].[Re].[Re] |
规范 SMILES |
[C-]#[O+].[C-]#[O+].[C-]#[O+].[C-]#[O+].[C-]#[O+].[C-]#[O+].[C-]#[O+].[C-]#[O+].[C-]#[O+].[C-]#[O+].[Re].[Re] |
Key on ui other cas no. |
14285-68-8 |
Pictograms |
Acute Toxic; Irritant |
产品来源 |
United States |
体外研究产品的免责声明和信息
请注意,BenchChem 上展示的所有文章和产品信息仅供信息参考。 BenchChem 上可购买的产品专为体外研究设计,这些研究在生物体外进行。体外研究,源自拉丁语 "in glass",涉及在受控实验室环境中使用细胞或组织进行的实验。重要的是要注意,这些产品没有被归类为药物或药品,他们没有得到 FDA 的批准,用于预防、治疗或治愈任何医疗状况、疾病或疾病。我们必须强调,将这些产品以任何形式引入人类或动物的身体都是法律严格禁止的。遵守这些指南对确保研究和实验的法律和道德标准的符合性至关重要。