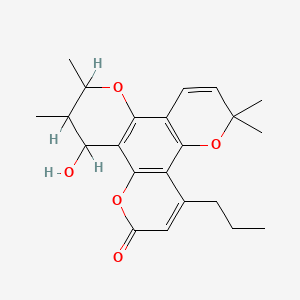
Calanolide
Overview
Description
Calanolide A is a naturally occurring pyranocoumarin first isolated from the tropical tree Calophyllum lanigerum. It exhibits dual activity as a non-nucleoside reverse transcriptase inhibitor (NNRTI) against HIV-1 and as an antimycobacterial agent against Mycobacterium tuberculosis (Mtb), including multidrug-resistant (MDR) and rifampin-resistant strains . Its enantiomer, (−)-calanolide A, is inactive, highlighting the critical role of stereochemistry in its biological activity . This compound B, a structural analog, shares similar anti-Mtb and anti-HIV-1 activity but is more abundant in natural sources like Calophyllum seed oil, offering cost-effective production advantages .
Key Pharmacological Properties of this compound A
- Mechanism: Inhibits HIV-1 reverse transcriptase (RT) by binding to an allosteric site distinct from nucleoside inhibitors . Against Mtb, it targets RNA synthesis but differs mechanistically from rifampin, as evidenced by its efficacy against rifampin-resistant strains .
- Pharmacokinetics: Oral bioavailability up to 32.7% in rats, brain and lymph tissue penetration, and a half-life of ~20 hours in humans at 800 mg doses . Common adverse effects include transient dizziness and an oily aftertaste .
Q & A
Basic Research Questions
Q. What are the established synthetic routes for Calanolide A, and how do researchers ensure enantiomeric purity?
this compound A is synthesized via multi-step processes, including the Pechmann reaction, Friedel-Crafts acylation, and Luche reduction, starting from phloroglucinol . To ensure enantiomeric purity, chiral separation techniques (e.g., chromatography using chiral stationary phases) are employed, as described in patent-derived methods for isolating racemic mixtures . Characterization via NMR, HPLC, and X-ray crystallography validates structural integrity .
Q. How does this compound A inhibit HIV-1 reverse transcriptase (RT), and what experimental assays confirm its mechanism?
this compound A acts as a non-nucleoside RT inhibitor (NNRTI), binding to the enzyme's allosteric site to disrupt viral replication. Researchers use in vitro RT inhibition assays (e.g., measuring IC₅₀ values) and molecular docking studies to map binding interactions. Synergistic effects with other antiretrovirals are tested in cell-based models .
Q. What structural features of this compound derivatives correlate with antiviral activity?
The cis-configuration of methyl groups at C-10 and C-11 is critical for potency, as shown in SAR studies. Modifications like 12-oxo derivatives reduce activity, while halogenation (e.g., 10-chloromethyl analogues) can enhance efficacy against resistant strains. Comparative crystallography of RT-Calanolide complexes identifies key hydrogen bonds and hydrophobic interactions .
Advanced Research Questions
Q. How can researchers address HIV-1 RT mutations (e.g., Y181C) that confer resistance to this compound analogues?
Resistance profiling involves engineering mutant RT enzymes via site-directed mutagenesis and testing inhibitory concentrations (IC₅₀) of novel analogues. For example, 10-chloromethyl-11-demethyl-12-oxo-Calanolide A shows retained efficacy against Y181C mutants, suggesting steric and electronic adjustments mitigate resistance . Combinatorial libraries and in silico saturation mutagenesis predict viable scaffolds .
Q. What methodologies optimize this compound pharmacokinetics (PK) for in vivo studies?
PK optimization includes pro-drug formulations (e.g., ester derivatives for enhanced bioavailability) and metabolic stability assays using liver microsomes. Phase 1 trials in healthy subjects assess parameters like Cₘₐₓ and t₁/₂, while compartmental modeling predicts tissue distribution .
Q. What challenges arise in scaling up this compound synthesis, and how are they mitigated?
Scalability issues include low yields in cyclization steps and racemization during chiral resolution. Flow chemistry and catalyst screening (e.g., asymmetric hydrogenation) improve efficiency. Process analytical technology (PAT) monitors critical quality attributes (e.g., enantiomeric excess) in real time .
Q. How do researchers design in vivo models to evaluate this compound’s antitumor potential?
Xenograft models (e.g., human tumor implants in immunodeficient mice) assess tumor regression and metastasis inhibition. Pharmacodynamic markers (e.g., apoptosis via TUNEL assays) and RNA-seq analysis identify pathway modulation. Dosing regimens are optimized using allometric scaling from in vitro EC₅₀ values .
Q. What statistical approaches reconcile contradictory efficacy data among this compound structural variants?
Multivariate analysis (e.g., principal component analysis) correlates structural descriptors (logP, polar surface area) with bioactivity. Meta-analyses of published IC₅₀ datasets identify outliers and adjust for assay variability (e.g., cell line differences) .
Q. How are clinical trials for this compound A structured to balance safety and pharmacokinetic endpoints?
Phase 1 trials use single ascending dose (SAD) designs with healthy volunteers. Endpoints include adverse event monitoring, plasma concentration-time curves, and metabolite profiling via LC-MS. Adaptive trial designs allow dose adjustments based on interim PK/PD modeling .
Q. What strategies validate this compound’s synergy with other antiretrovirals in combination therapy?
Isobologram analysis and Chou-Talalay combination indices quantify synergistic, additive, or antagonistic effects. In vitro models (e.g., PBMC cultures infected with HIV-1) test viral load reduction under combination regimens. Resistance selection experiments assess barrier to cross-resistance .
Comparison with Similar Compounds
Structural Analogues and Stereochemical Variations
Calanolides are defined by a tetracyclic coumarin core (rings A–D). Modifications to chiral centers (C-10, C-11, C-12) and ring systems significantly alter activity:
Notes:
- Stereochemistry: The 10R/11S/12S configuration in (+)-calanolide A is essential for RT inhibition. Inversion at C-10 or C-11 (e.g., pseudothis compound C) abolishes activity .
- C-Ring Modifications: Derivatives like GUT-70, which lack the pyran ring (C-ring), retain nanomolar potency by targeting viral entry and NF-κB signaling .
Pharmacokinetic Comparison
(+)-Dihydrothis compound A, a hydrogenated derivative, shows improved metabolic stability and is prioritized for preclinical development .
Resistance Profiles
- HIV-1: (+)-Calanolide A retains activity against the Y181C RT mutation (common in NNRTI resistance) but shows 30-fold reduced efficacy against Y188H mutants .
- Mtb: Active against rifampin- and streptomycin-resistant strains, suggesting a novel target distinct from RNA polymerase .
Structure-Activity Relationships (SAR)
- Ring D : The 12β-hydroxyl group is critical for RT inhibition. Its removal or oxidation reduces potency .
- Chiral Centers : Reduction of chiral centers (e.g., 11-demethyl-12-oxo derivatives) maintains activity, simplifying synthesis .
Therapeutic Potential and Challenges
- Advantages : Dual HIV/Mtb activity, low toxicity (TI > 1000 for some derivatives), and oral dosing .
- Limitations: Natural scarcity of (+)-calanolide A (resolved via synthetic routes ), and moderate bioavailability in humans .
- Future Directions: Dihydrothis compound A and C-ring-modified analogs (e.g., GUT-70) are under investigation for improved pharmacokinetics and resistance profiles .
Preparation Methods
Natural Extraction and Isolation of Calanolides
Calanolides were first isolated from the tropical rainforest tree Calophyllum lanigerum var. austrocoriaceum in Sarawak, Malaysia. The extraction process involves sequential solvent partitioning and chromatographic purification .
Solvent Extraction and Partitioning
Dried fruits and twigs are ground and extracted with a 1:1 mixture of dichloromethane and methanol. The crude extract undergoes sequential partitioning with n-hexane, carbon tetrachloride, and ethyl acetate. Bioactivity-guided fractionation identifies the n-hexane and CCl₄ fractions as enriched in calanolides .
Chromatographic Purification
Vacuum liquid chromatography (VLC) on silica gel with a gradient of n-hexane/ethyl acetate separates calanolide precursors. Final purification employs high-performance liquid chromatography (HPLC):
-
Normal-phase HPLC : Resolves this compound A (1), this compound B (4), and pseudothis compound D (9).
-
Reversed-phase HPLC : Isolates 12-acetoxythis compound A (2) and 12-methoxy derivatives .
Typical yields from natural sources remain low (0.001–0.01% w/w), necessitating synthetic approaches for scalable production .
Synthetic Routes to Racemic this compound A
The first total synthesis of (±)-calanolide A, reported in U.S. Patent 5,489,697, established a five-step route from phloroglucinol and ethyl butyrylacetate .
Pechmann Condensation
5,7-Dihydroxy-4-propylcoumarin (2) is synthesized via acid-catalyzed condensation:
2\text{SO}4} \text{5,7-Dihydroxy-4-propylcoumarin (2)} \quad
This step achieves quantitative yield under reflux conditions .
Acylation and Cyclization
Coumarin 2 undergoes Friedel-Crafts acylation with propionyl chloride/AlCl₃ to install the C-8 propionyl group, yielding 4-propyl-5,7-dihydroxy-8-propionylcoumarin (3). Reaction with 4,4-dimethoxy-2-methylbutan-2-ol forms the pyran ring (chromene 4 ) .
Robinson-Kostanecki Reaction and Reduction
Chromene 4 is converted to enone 5 via Robinson-Kostanecki reaction (65% yield). Subsequent Luche reduction (NaBH₄/CeCl₃) stereoselectively produces (±)-calanolide A (94% yield) :
4/\text{CeCl}3} \text{(±)-Calanolide A} \quad
Enantioselective Synthesis and Resolution
Racemic this compound A resolution exploits enzymatic acylation for chiral separation .
Enzymatic Kinetic Resolution
Racemic aldol intermediates (e.g., (±)-8a ) are treated with lipases (e.g., Pseudomonas cepacia), selectively acylating the (−)-enantiomer. Chromatographic separation yields enantiopure (+)-calanolide A (ee >98%) .
Mitsunobu Reaction for cis-Dihydroxylation
The Mitsunobu reaction (DEAD/PPh₃) converts secondary alcohols to cis-diols, enabling synthesis of this compound C from (+)-8b .
Biosynthetic Pathways
This compound biosynthesis in Calophyllum species proceeds via phenylpropanoid and polyketide pathways .
Coumarin Backbone Formation
-
Phenylpropanoid pathway : trans-Cinnamic acid → p-coumaric acid → 2-hydroxy-p-coumaric acid (mediated by cinnamate 4-hydroxylase).
-
Polyketide extension : 4-Coumaroyl-CoA undergoes chain elongation to form the 3-propyl sidechain .
Cyclization and Modifications
A proposed Wagner-Meerwein rearrangement forms the pyran ring, followed by P450-mediated oxidation to install hydroxyl groups at C-12 and C-11 .
Modern Analog Synthesis and Derivatives
Recent work focuses on simplifying the this compound scaffold while retaining anti-HIV activity .
Ring C-Opened Analogues
Replacing the C ring with a thiazole or oxadiazole moiety reduces stereochemical complexity. Compound 4b (70% yield) shows EC₅₀ = 0.82 μM against HIV-1 :
Microwave-Assisted Synthesis
Microwave irradiation reduces reaction times from hours to minutes. For example, cyclocondensation of 3 with hydrazines achieves 85% yield in 15 min vs. 12 h conventionally .
Industrial-Scale Production Challenges
Solvent Recovery Systems
Large-scale synthesis (Patent WO1998038193A1) employs closed-loop solvent recovery to minimize waste from acetonitrile and dichloromethane .
Crystallization Optimization
Seeding protocols control polymorphism during final crystallization, ensuring consistent melting points (m.p. 184–186°C for 4b ) .
Properties
Molecular Formula |
C22H26O5 |
---|---|
Molecular Weight |
370.4 g/mol |
IUPAC Name |
18-hydroxy-10,10,16,17-tetramethyl-6-propyl-3,9,15-trioxatetracyclo[12.4.0.02,7.08,13]octadeca-1(14),2(7),5,8(13),11-pentaen-4-one |
InChI |
InChI=1S/C22H26O5/c1-6-7-13-10-15(23)26-21-16(13)20-14(8-9-22(4,5)27-20)19-17(21)18(24)11(2)12(3)25-19/h8-12,18,24H,6-7H2,1-5H3 |
InChI Key |
NIDRYBLTWYFCFV-UHFFFAOYSA-N |
SMILES |
CCCC1=CC(=O)OC2=C1C3=C(C=CC(O3)(C)C)C4=C2C(C(C(O4)C)C)O |
Canonical SMILES |
CCCC1=CC(=O)OC2=C1C3=C(C=CC(O3)(C)C)C4=C2C(C(C(O4)C)C)O |
Origin of Product |
United States |
Synthesis routes and methods I
Procedure details
Synthesis routes and methods II
Procedure details
Retrosynthesis Analysis
AI-Powered Synthesis Planning: Our tool employs the Template_relevance Pistachio, Template_relevance Bkms_metabolic, Template_relevance Pistachio_ringbreaker, Template_relevance Reaxys, Template_relevance Reaxys_biocatalysis model, leveraging a vast database of chemical reactions to predict feasible synthetic routes.
One-Step Synthesis Focus: Specifically designed for one-step synthesis, it provides concise and direct routes for your target compounds, streamlining the synthesis process.
Accurate Predictions: Utilizing the extensive PISTACHIO, BKMS_METABOLIC, PISTACHIO_RINGBREAKER, REAXYS, REAXYS_BIOCATALYSIS database, our tool offers high-accuracy predictions, reflecting the latest in chemical research and data.
Strategy Settings
Precursor scoring | Relevance Heuristic |
---|---|
Min. plausibility | 0.01 |
Model | Template_relevance |
Template Set | Pistachio/Bkms_metabolic/Pistachio_ringbreaker/Reaxys/Reaxys_biocatalysis |
Top-N result to add to graph | 6 |
Feasible Synthetic Routes
Disclaimer and Information on In-Vitro Research Products
Please be aware that all articles and product information presented on BenchChem are intended solely for informational purposes. The products available for purchase on BenchChem are specifically designed for in-vitro studies, which are conducted outside of living organisms. In-vitro studies, derived from the Latin term "in glass," involve experiments performed in controlled laboratory settings using cells or tissues. It is important to note that these products are not categorized as medicines or drugs, and they have not received approval from the FDA for the prevention, treatment, or cure of any medical condition, ailment, or disease. We must emphasize that any form of bodily introduction of these products into humans or animals is strictly prohibited by law. It is essential to adhere to these guidelines to ensure compliance with legal and ethical standards in research and experimentation.