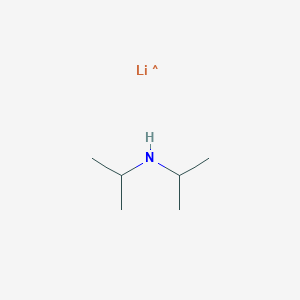
Lithium diisopropylamine
- Click on QUICK INQUIRY to receive a quote from our team of experts.
- With the quality product at a COMPETITIVE price, you can focus more on your research.
Overview
Description
Lithium diisopropylamide (LDA), with the chemical formula C₆H₁₄LiN, is a sterically hindered, strong non-nucleophilic base widely used in deprotonation reactions and organometallic synthesis . It is commercially available as a solution in tetrahydrofuran (THF)/hexanes/ethylbenzene but is pyrophoric in its solid form . Due to instability during prolonged storage, LDA is often prepared in situ via the reaction of diisopropylamine with n-butyllithium (n-BuLi) . Its high basicity (estimated pKa ~36–40 in THF) and bulky isopropyl substituents make it ideal for regioselective deprotonation of weakly acidic substrates (e.g., ketones, esters, and aromatic heterocycles) .
Scientific Research Applications
Deprotonation Reactions
Lithium diisopropylamine is widely utilized for the deprotonation of various substrates, enabling the formation of nucleophilic species that can participate in further reactions. Notably, it effectively deprotonates esters at the α-position without attacking the carbonyl group. This selectivity is crucial for synthesizing complex molecules .
Table 1: Common Substrates Deprotonated by this compound
Substrate Type | Example Compound | Reaction Type |
---|---|---|
Carbon Acids | Acetic Acid | α-Deprotonation |
Esters | Ethyl Acetate | α-Deprotonation |
Alcohols | Ethanol | Deprotonation |
Lithiation of Aromatic Compounds
This compound facilitates the lithiation of aromatic compounds, which is essential for subsequent coupling reactions. The regioselectivity observed during these lithiation reactions can vary significantly based on the source and preparation method of LDA. For instance, commercially available LDA shows different reactivity compared to LDA prepared from n-butyllithium and diisopropylamine .
Case Study: Ortholithiation of Fluoropyridines
In a study examining the ortholithiation of 2-fluoropyridine using this compound, it was found that the reaction proceeds at low temperatures (-78 °C) and exhibits complex kinetics influenced by both autocatalysis and trace contaminants such as lithium chloride . The study highlighted the importance of reaction conditions and LDA's aggregation state on the outcome.
Reaction Mechanisms
The mechanisms underlying LDA-mediated reactions are intricate and often involve dimerization and aggregation phenomena. For example, during the lithiation process, LDA can exist in various oligomeric states depending on solvent polarity and temperature, affecting its reactivity .
Table 2: Aggregation States of this compound in Different Solvents
Solvent Type | Aggregation State | Temperature Range |
---|---|---|
Tetrahydrofuran | Dimer | -78 °C |
Toluene | Trimer/Tetramer | Room Temperature |
Autocatalysis and Rate Limiting Steps
Studies have shown that certain LDA-mediated reactions exhibit autocatalytic behavior, where products formed during the reaction can catalyze further transformations. This phenomenon complicates kinetic analyses but provides insights into optimizing reaction conditions for improved yields .
Industrial Applications
Beyond laboratory synthesis, this compound has significant industrial applications:
- Pharmaceuticals : LDA is used in synthesizing various pharmaceutical compounds due to its ability to create reactive intermediates.
- Agricultural Chemicals : It serves as a precursor in synthesizing herbicides and other agrochemicals.
Chemical Reactions Analysis
Deprotonation and Lithiation Reactions
LDA selectively deprotonates acidic substrates such as carbonyl compounds, nitriles, and aromatics. Key findings include:
-
Ortholithiation of 2,6-difluoropyridine :
LDA mediates the conversion of 2,6-difluoropyridine (1 ) to aryllithium intermediate 3 at −78°C, followed by nucleophilic attack at 0°C to form aminopyridine 2 (Scheme 1) . Rate studies support a reversible lithiation mechanism (eq 1) over pyridyne intermediates . -
1,4-Dichloro-2,5-dimethoxybenzene lithiation :
LDA deprotonates this arene via a cascade involving dimeric LDA deaggregation. Computational studies reveal rate-limiting transition states (e.g., 4 ) and autocatalysis by aryllithium products (Scheme 1) .
Substitution Reactions
LDA participates in nucleophilic substitutions under controlled conditions:
-
Fluoride displacement : In the reaction of 2,6-difluoropyridine with LDA, LiF elimination is kinetically disfavored compared to direct substitution. Zeroth-order dependence on diisopropylamine confirms a non-pyridyne pathway .
Aggregation and Solvent Effects
LDA’s reactivity is intricately tied to its solvation state:
-
THF coordination :
-
In THF, LDA exists as solvated dimers (e.g., [Li₂(NiPr₂)₂(THF)ₓ]) .
-
Excess THF (>1 equiv per LDA) destabilizes the base via THF metalation, leading to decomposition .
THF/LDA Ratio Stability at 20.5°C Decomposition Rate 0.5–1.0 Stable (>30 days) <5% activity loss 2.0 Unstable 15% loss in 7 days 6.4 Rapid decomposition 100% loss in 7 days -
-
HMPA cosolvent :
Hexamethylphosphoramide (HMPA) accelerates reactions by promoting tetrasolvated dimer transition states (e.g., 16 ) or triple-ion intermediates (17 ), as seen in conjugate additions to unsaturated esters .
Preparation and Stabilization
Synthetic protocols impact LDA’s performance:
-
Direct synthesis from lithium metal :
Reacting Li metal with diisopropylamine and styrene/isoprene in THF (≤2 mol THF per electron carrier) yields stable, non-pyrophoric LDA solutions .Electron Carrier Byproduct Boiling Point Preferred Use Case Styrene Ethylbenzene 136°C High-boiling products Isoprene 2-Methyl-2-butene 36°C Low-boiling products
Kinetic Isotope Effects (KIEs)
Deuterium labeling reveals mechanistic nuances:
-
Ortholithiation of deuterated arenes :
Perdeuteration of substrates lowers zero-point energy (ZPE) barriers, unmasking competing dimer-based pathways (e.g., 13 vs. 16 ) . Rate laws transition from first-order to zeroth-order in substrate under high deuteration .
Catalytic Effects of LiCl
LiCl alters reaction coordinates:
-
Accelerated lithiation :
Traces of LiCl catalyze LDA-mediated reactions via mixed-aggregate transition states (e.g., 5 ), bypassing rate-limiting deaggregation steps .
Conjugate Additions
LDA/HMPA mixtures enable 1,4-additions:
Q & A
Basic Research Questions
Q. How is lithium diisopropylamide (LDA) synthesized, and what factors influence its purity and reactivity?
LDA is typically synthesized by reacting diisopropylamine with an alkyllithium reagent (e.g., n-butyllithium) in anhydrous tetrahydrofuran (THF) or diethyl ether at low temperatures (0°C to -78°C) under inert conditions . Key factors include:
- Stoichiometry : A 1:1 molar ratio of diisopropylamine to alkyllithium ensures complete deprotonation .
- Solvent choice : THF enhances solubility and stabilizes the LDA dimer, while ethereal solvents favor monomeric forms, affecting reactivity .
- Temperature control : Low temperatures (-78°C) minimize side reactions, such as ligand redistribution or decomposition .
Q. What are the primary applications of LDA in organic synthesis?
LDA is a strong, non-nucleophilic base widely used for:
- Enolate formation : Deprotonates carbonyl compounds (ketones, esters) regioselectively under kinetic control, enabling crossed aldol and alkylation reactions .
- Directed ortho-lithiation : Activates aromatic substrates (e.g., halogenated arenes) for functionalization at specific positions .
- Anionic polymerization : Initiates controlled polymerization of styrenes and acrylates in polymer chemistry .
Q. How does solvent selection impact LDA-mediated reactions?
Solvents modulate LDA’s aggregation state and reactivity:
- THF : Stabilizes dimeric or trimeric aggregates, slowing reaction kinetics but improving selectivity .
- Diethyl ether : Favors monomeric LDA, increasing base strength and reaction rates .
- Hexamethylphosphoramide (HMPA) : Added as a cosolvent to enhance solubility and stabilize transition states in challenging deprotonations .
Q. What safety protocols are critical for handling LDA?
Key safety measures include:
- Inert atmosphere : Use argon or nitrogen to prevent moisture/oxygen exposure .
- Temperature control : Avoid exothermic decomposition by maintaining reactions below -30°C .
- Quenching protocols : Neutralize excess LDA with chilled ethanol or isopropanol before disposal .
Advanced Research Questions
Q. How does LiCl influence the regioselectivity of LDA-mediated ortho-lithiation?
Trace LiCl (≤100 ppm) alters reaction pathways:
- Autocatalysis : LiCl accelerates lithiation by stabilizing monomeric LDA species, enabling selective 2-position metalation in halogenated arenes .
- Reversibility : LiCl promotes equilibration between lithiated intermediates, favoring thermodynamically stable products .
- Kinetic vs. thermodynamic control : LiCl-free conditions favor irreversible, kinetically controlled products, while LiCl enables reversible pathways .
Q. What mechanistic insights explain LDA’s role in β-ketocarboxylate reactions?
LDA reacts with β-ketocarboxylates via:
- Disproportionation : Forms enolate intermediates that undergo decarboxylation or alkylation, depending on electrophile choice (e.g., alkyl halides vs. CO₂) .
- Interference mitigation : Diisopropylamine (a byproduct) must be minimized, as it competes for carboxylation sites .
Q. How do aggregation states of LDA affect its reactivity in low-concentration ligand systems?
At substoichiometric ligand concentrations (e.g., <1 equiv. THF):
- Partially solvated oligomers : Dimers and trimers dominate, reducing base strength but enabling unique reactivity in sterically hindered systems .
- Solvent-free conditions : Enhance electrophilic trapping by reducing solvation barriers, useful in large-scale syntheses .
Q. What strategies prevent side reactions during LDA-mediated enolate formation?
Mitigation approaches include:
- Precooling reagents : Substrates and LDA solutions are cooled to -78°C to suppress aldol condensation or over-alkylation .
- Controlled stoichiometry : Use 1.1–1.2 equiv. LDA to avoid excess base-induced decomposition .
- In situ quenching : Immediate electrophile addition after enolate formation prevents retro-aldol reactions .
Q. How is LDA employed in stereocontrolled total syntheses?
Case studies include:
- Lyconadin A/B synthesis : LDA generates key enolate intermediates for cyclization, with stereochemistry controlled by chiral auxiliaries .
- Tochuinyl acetate synthesis : LDA-mediated alkylation of cyclopentane derivatives achieves high diastereoselectivity via chelation control .
Q. Methodological Considerations
- Data contradiction resolution : Conflicting reports on LDA’s aggregation (e.g., dimer vs. trimer dominance) often arise from solvent purity or LiCl contamination . Validate aggregation states via ⁶Li/¹⁵N NMR .
- Reaction optimization : Use kinetic profiling (e.g., in situ IR) to identify rate-limiting steps in lithiation reactions .
Comparison with Similar Compounds
Comparison with Similar Compounds
Lithium vs. Sodium Diisopropylamide
Key Differences :
- Synthesis : NaDA requires sodium metal and isoprene, making it less convenient than LDA’s one-step preparation from n-BuLi .
- Reactivity : NaDA may outperform LDA in reactions demanding extreme basicity but lacks LDA’s widespread use due to handling challenges .
Comparison with Other Lithium Amides
Lithium Hexamethyldisilazide (LiHMDS)
Key Insight : LDA’s steric bulk enables selective deprotonation of hindered substrates, whereas LiHMDS is preferred for less acidic protons or silylations .
Lithium Dialkylamides (e.g., Lithium Dimethylamide)
- Basicity : Smaller substituents (e.g., methyl) reduce basicity. LDA’s isopropyl groups enhance both basicity and selectivity .
- Reaction Efficiency: In HMPA complexes, LDA outperforms lithium dimethylamide in reactions like ketone enolization, yielding 39% vs. 10% product .
Comparison with Alkyllithium Reagents (e.g., n-BuLi)
Key Insight : While n-BuLi is stronger, its nucleophilicity limits selectivity. LDA’s steric hindrance prevents unwanted nucleophilic attacks, making it superior for deprotonation .
Potassium and Heavier Alkali Metal Amides
- Synthesis : Potassium diisopropylamide (KDA) is prepared in situ via LDA + t-BuOK, but its use is rare .
Solvent and Aggregation Effects
LDA’s reactivity is influenced by solvent and aggregation state:
Properties
Molecular Formula |
C6H15LiN |
---|---|
Molecular Weight |
108.2 g/mol |
InChI |
InChI=1S/C6H15N.Li/c1-5(2)7-6(3)4;/h5-7H,1-4H3; |
InChI Key |
OVEHNNQXLPJPPL-UHFFFAOYSA-N |
Canonical SMILES |
[Li].CC(C)NC(C)C |
Origin of Product |
United States |
Disclaimer and Information on In-Vitro Research Products
Please be aware that all articles and product information presented on BenchChem are intended solely for informational purposes. The products available for purchase on BenchChem are specifically designed for in-vitro studies, which are conducted outside of living organisms. In-vitro studies, derived from the Latin term "in glass," involve experiments performed in controlled laboratory settings using cells or tissues. It is important to note that these products are not categorized as medicines or drugs, and they have not received approval from the FDA for the prevention, treatment, or cure of any medical condition, ailment, or disease. We must emphasize that any form of bodily introduction of these products into humans or animals is strictly prohibited by law. It is essential to adhere to these guidelines to ensure compliance with legal and ethical standards in research and experimentation.