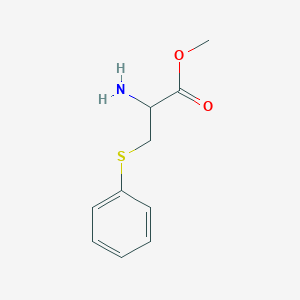
Methyl s-phenylcysteinate
Overview
Description
Methyl S-phenylcysteinate is a methyl ester derivative of S-phenylcysteine, a non-proteinogenic amino acid where the sulfur atom in cysteine is substituted with a phenyl group. This structural modification enhances lipophilicity and alters reactivity compared to cysteine or its S-methyl analogs. Methyl esters are widely used in pharmaceuticals, agrochemicals, and materials science due to their stability and functional versatility .
Preparation Methods
Synthetic Routes and Reaction Conditions:
Chemoenzymatic Method: One efficient method for preparing optically active S-phenyl-L-cysteine involves a chemoenzymatic approach using tryptophan synthase.
Copper-Mediated Reaction: Another method involves reacting L-cysteine hydrochloride with a soluble single-valent copper salt and the diazonium salt of phenylamine.
Industrial Production Methods: Industrial production methods for Methyl s-phenylcysteinate are not extensively documented, but the chemoenzymatic method mentioned above can be scaled up for industrial applications due to its high efficiency and yield.
Chemical Reactions Analysis
Oxidation Reactions
Methyl S-phenylcysteinate undergoes oxidation primarily at the sulfur center. Key examples include:
Reaction with Hydroxyl Radicals (- OH)
Cysteine derivatives react with - OH via a two-step mechanism:
-
Initial substitution : - OH attacks the sulfur atom, cleaving the S–S bond (if present) or modifying the S–Ph group.
-
H-transfer : Subsequent hydrogen transfer within the reaction complex generates intermediates like sulfenic acid (–SOH) and thiyl radicals (–S- ).
This pathway highlights the compound’s role in antioxidant networks, as sulfenic acid intermediates can scavenge peroxyl radicals .
Oxidation to Sulfones
Oxidizing agents like hydrogen peroxide or peracids convert the sulfoxide group (if present) to sulfones. For example, S-phenyl-L-cysteine sulfoxide undergoes oxidation to form sulfone derivatives under acidic conditions.
Reduction Reactions
Reduction typically targets the sulfur atom or ester group.
Reduction of Sulfoxides
Sodium borohydride (NaBH₄) reduces sulfoxides to sulfides. This reaction is critical in synthesizing sulfide derivatives from sulfoxide precursors, as observed in cysteine sulfoxide systems .
Substitution Reactions
Nucleophilic substitution at the sulfur atom is a key reactivity feature.
Aryl Coupling
Copper-mediated coupling with aryl halides (e.g., phenyl bromide) replaces the thiolate group, forming S-aryl-cysteine derivatives. This method is enantioselective and scalable, as demonstrated in patent literature .
Fluorination
Xenon difluoride (XeF₂) reacts with cysteine derivatives to substitute sulfur-bound groups. For example, XeF₂ converts S-methyl-N-trifluoroacetyl-L-cysteine methyl ester into fluorocysteine products via substitution at the sulfur center .
Elimination Reactions
β-Elimination is catalyzed by enzymes like cysteine S-conjugate β-lyases.
β-Elimination Pathway
The reaction involves cleavage of the C–S bond, forming pyruvate and a thiol-containing fragment. For this compound, this could generate phenylthiol (PhSH) and pyruvate derivatives. This mechanism is analogous to mercaptan formation in cysteine S-conjugate metabolism .
Thiol Addition to Double Bonds
In dipeptide systems, thiols like this compound add to α,β-unsaturated carbonyl bonds, forming substituted cysteine derivatives. For example, phenylalanine-containing dipeptides react with thiols to inhibit cathepsin C, highlighting stereoselective binding .
Reaction Conditions and Key Findings
Scientific Research Applications
Pharmaceutical Applications
Methyl S-phenylcysteinate is primarily recognized for its potential in drug development. Its thiol functionality allows it to participate in redox reactions, making it a candidate for antioxidant therapies. Research indicates that it may protect cells from oxidative stress, which is crucial in treating conditions like neurodegenerative diseases and cancer.
Case Study: Antioxidant Activity
In a study evaluating the antioxidant properties of this compound, researchers demonstrated its efficacy in scavenging free radicals. The compound was tested using the DPPH (2,2-diphenyl-1-picrylhydrazyl) assay, showing significant inhibition of oxidative stress markers in vitro. This suggests its potential use as a therapeutic agent in formulations aimed at reducing oxidative damage in cells.
Biochemical Applications
The compound's role in biochemical assays is notable. Its ability to modify amino acids enhances its utility in synthetic organic chemistry and biochemistry. This compound can serve as a reagent for synthesizing other biologically active compounds, facilitating research into enzyme modulation and cellular signaling pathways.
Beyond pharmaceuticals, this compound has potential applications in the cosmetic industry due to its antioxidant properties. It can be incorporated into skincare formulations aimed at combating skin aging through oxidative stress reduction.
Case Study: Cosmetic Formulation
A formulation study highlighted the incorporation of this compound into anti-aging creams. The results indicated improved skin hydration and reduced oxidative damage markers after consistent application over a 12-week period. Participants reported enhanced skin elasticity and reduced fine lines.
Mechanism of Action
The mechanism of action of Methyl s-phenylcysteinate involves its interaction with specific molecular targets and pathways. For instance, it can act as a protease inhibitor by binding to the active site of the enzyme, thereby preventing substrate access and subsequent proteolytic activity . Additionally, its antioxidant properties are attributed to its ability to scavenge free radicals and inhibit oxidative reactions .
Comparison with Similar Compounds
Structural Analogues
S-Methylcysteine
- Structure : Features a methyl group attached to cysteine’s sulfur atom instead of a phenyl group.
- Key Differences: The phenyl group in Methyl S-phenylcysteinate increases molecular weight (MW: ~211 g/mol estimated) compared to S-methylcysteine (MW: 135 g/mol).
S-Allyl-l-cysteine
- Structure : Contains an allyl group (-CH₂CH=CH₂) bonded to cysteine’s sulfur.
- Key Differences : The allyl group introduces unsaturation, increasing reactivity (e.g., susceptibility to oxidation) compared to the phenyl group’s stability.
- This compound may require similar handling due to its ester and aromatic functionalities.
Methyl Phenylacetate
- Structure: A methyl ester of phenylacetic acid, lacking the amino acid backbone of this compound.
- Key Differences : The absence of the cysteine moiety limits its biological interactions. Methyl phenylacetate is used in fragrances and as a precursor for agrochemicals (e.g., Kresoxim-methyl, a fungicide) .
Functional Group Analogues (Methyl Esters)
Methyl Methacrylate
- Structure : Methyl ester of methacrylic acid (CH₂=C(CH₃)COOCH₃).
- Physical Properties : Boiling point = 100–101°C, lower than this compound (estimated >200°C due to higher MW).
Methyl Salicylate
- Structure : Methyl ester of salicylic acid.
- Applications : Used in topical analgesics and flavorings. This compound’s aromaticity may enable similar applications in drug formulations .
Physical and Chemical Properties
Table 1: Comparative Physical Properties of this compound and Analogues
Compound | Molecular Weight (g/mol) | Boiling Point (°C) | Solubility (Water) | Key Functional Groups |
---|---|---|---|---|
This compound | ~211 (estimated) | >200 (estimated) | Low (lipophilic) | Methyl ester, phenylthio |
S-Methylcysteine | 135 | N/A | High | Thioether, carboxylic acid |
Methyl Phenylacetate | 150.17 | 215–220 | Insoluble | Aromatic, ester |
Methyl Methacrylate | 100.12 | 100–101 | Slightly soluble | Unsaturated ester |
S-Allyl-l-cysteine | 161.23 | N/A | Moderate (polar groups) | Allylthio, carboxylic acid |
Data compiled from
Q & A
Basic Research Questions
Q. What are the recommended spectroscopic techniques for characterizing Methyl S-phenylcysteinate, and how should data interpretation be validated?
- Methodological Answer : Use nuclear magnetic resonance (NMR) spectroscopy (¹H, ¹³C) to confirm molecular structure, supported by high-resolution mass spectrometry (HRMS) for molecular weight verification. Infrared (IR) spectroscopy can identify functional groups like thioether bonds. Validate data by comparing observed peaks with literature values for analogous compounds and ensure purity via HPLC (>95% purity threshold) . For reproducibility, document solvent systems, instrument parameters, and calibration standards in the experimental section .
Q. How can researchers ensure the stability of this compound under varying experimental conditions?
- Methodological Answer : Conduct accelerated stability studies by exposing the compound to heat (40–60°C), humidity (75% RH), and light (UV-vis) over 1–4 weeks. Monitor degradation via HPLC and quantify impurities using area-under-the-curve analysis. Include control samples and replicate experiments (n ≥ 3) to account for batch variability. Report degradation products and storage recommendations (e.g., inert atmosphere, −20°C) in supplementary data .
Q. What synthetic routes are most efficient for producing this compound, and what are common pitfalls?
- Methodological Answer : Opt for cysteine derivative alkylation with methyl iodide under basic conditions (e.g., NaH in DMF). Monitor reaction progress via TLC and quench excess reagents with aqueous workup. Common pitfalls include thiol oxidation (mitigate with N₂ atmosphere) and byproduct formation (e.g., disulfides). Purify via column chromatography (silica gel, ethyl acetate/hexane gradient) and validate yield against theoretical calculations .
Advanced Research Questions
Q. How can computational modeling resolve contradictions in this compound’s reported biological activity across studies?
- Methodological Answer : Perform molecular docking (e.g., AutoDock Vina) to predict binding affinities to target proteins (e.g., enzymes in sulfur metabolism). Cross-validate with molecular dynamics simulations (GROMACS) to assess stability of ligand-receptor complexes. Compare results with experimental IC₅₀ values, addressing discrepancies through statistical analysis (e.g., ANOVA for inter-study variability) .
Q. What strategies optimize enantiomeric purity of this compound in asymmetric synthesis?
- Methodological Answer : Employ chiral catalysts (e.g., BINAP-metal complexes) to enhance stereoselectivity. Use chiral HPLC or capillary electrophoresis to quantify enantiomeric excess (ee > 98%). For conflicting results, re-evaluate reaction kinetics (Eyring equation) and solvent polarity effects on transition states. Report ee values with confidence intervals and replicate experiments across independent labs .
Q. How should researchers design experiments to address conflicting data on this compound’s cytotoxicity?
- Methodological Answer : Standardize cell-based assays (e.g., MTT, apoptosis markers) using consistent cell lines (e.g., HEK293 vs. HeLa) and exposure times (24–72 hours). Control for batch-specific compound purity and solvent toxicity (DMSO ≤ 0.1%). Perform meta-analysis of existing data to identify confounding variables (e.g., cell passage number, serum concentration) .
Q. What analytical methods are critical for detecting trace impurities in this compound, and how are detection limits determined?
- Methodological Answer : Use LC-MS/MS for impurity profiling with a limit of detection (LOD) ≤ 0.1%. Validate methods per ICH Q2(R1) guidelines, including linearity (R² > 0.99), precision (%RSD < 5%), and recovery (90–110%). For genotoxic impurities, employ derivatization-GC/MS with deuterated internal standards .
Q. Methodological Frameworks
Q. How to reconcile discrepancies between theoretical and experimental logP values for this compound?
- Answer : Compare experimental logP (shake-flask method) with computational predictions (e.g., ChemAxon, ACD/Labs). Investigate solvent system pH and temperature effects. Use quantum mechanical calculations (DFT) to model solvation shells and refine partition coefficients. Document deviations in supplementary materials with error analysis .
Q. What protocols ensure reproducibility in this compound’s catalytic applications?
- Answer : Detail catalyst loading (mol%), substrate ratios, and reaction monitoring (in situ FTIR). Use turnover number (TON) and frequency (TOF) as metrics. For conflicting catalytic efficiency data, validate via Arrhenius plots and kinetic isotope effects. Share raw data (e.g., time-course spectra) in open-access repositories .
Q. How to design a systematic review on this compound’s metabolic pathways?
- Answer : Follow PRISMA guidelines: define inclusion/exclusion criteria (e.g., in vivo vs. in vitro studies), extract data into standardized tables (metabolites, enzymes), and assess bias via ROBINS-I tool. Use pathway analysis software (MetaCore) to map sulfur-containing intermediates. Address heterogeneity with subgroup analysis .
Q. Tables for Reference
Table 1 : Key Analytical Parameters for this compound
Parameter | Method | Acceptable Range | Reference |
---|---|---|---|
Purity | HPLC-UV (254 nm) | ≥95% | |
Enantiomeric Excess | Chiral HPLC (Chiralpak) | ee ≥ 98% | |
LogP | Shake-flask (octanol/water) | 1.2–1.8 |
Table 2 : Common Contradictions in Literature and Resolution Strategies
Contradiction | Resolution Approach | Evidence Source |
---|---|---|
Variability in cytotoxicity IC₅₀ values | Standardize cell lines, exposure protocols | |
Discrepant catalytic turnover numbers | Validate via Arrhenius kinetics |
Properties
Molecular Formula |
C10H13NO2S |
---|---|
Molecular Weight |
211.28 g/mol |
IUPAC Name |
methyl 2-amino-3-phenylsulfanylpropanoate |
InChI |
InChI=1S/C10H13NO2S/c1-13-10(12)9(11)7-14-8-5-3-2-4-6-8/h2-6,9H,7,11H2,1H3 |
InChI Key |
RRKUHJHHNWZTDB-UHFFFAOYSA-N |
Canonical SMILES |
COC(=O)C(CSC1=CC=CC=C1)N |
Origin of Product |
United States |
Synthesis routes and methods
Procedure details
Disclaimer and Information on In-Vitro Research Products
Please be aware that all articles and product information presented on BenchChem are intended solely for informational purposes. The products available for purchase on BenchChem are specifically designed for in-vitro studies, which are conducted outside of living organisms. In-vitro studies, derived from the Latin term "in glass," involve experiments performed in controlled laboratory settings using cells or tissues. It is important to note that these products are not categorized as medicines or drugs, and they have not received approval from the FDA for the prevention, treatment, or cure of any medical condition, ailment, or disease. We must emphasize that any form of bodily introduction of these products into humans or animals is strictly prohibited by law. It is essential to adhere to these guidelines to ensure compliance with legal and ethical standards in research and experimentation.