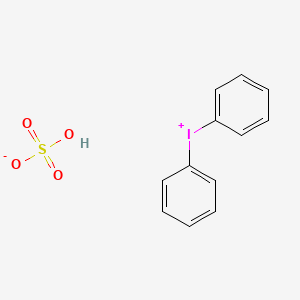
Diphenyliodonium hydrogen sulfate
- Click on QUICK INQUIRY to receive a quote from our team of experts.
- With the quality product at a COMPETITIVE price, you can focus more on your research.
Overview
Description
These salts are widely recognized for their strong oxidizing properties and versatility in organic synthesis, photopolymerization, and biochemical applications. The iodonium core (Ar₂I⁺) facilitates electron-transfer reactions, enabling its use as a photoinitiator in resins and an inhibitor of flavoenzymes such as NADPH oxidase . The hydrogen sulfate counterion enhances solubility in polar solvents, making it suitable for aqueous-phase reactions and biological studies .
Scientific Research Applications
Inhibition of Hydrogen Sulfide Production
One of the primary applications of diphenyliodonium hydrogen sulfate is in the oil and gas industry, where it serves as an effective inhibitor of hydrogen sulfide production. Hydrogen sulfide is a toxic gas that poses significant risks in hydrocarbon extraction processes. Diphenyliodonium salts have been shown to inhibit the growth of sulfur-utilizing prokaryotes, thereby reducing hydrogen sulfide concentrations in hydrocarbon systems.
Case Study: Microbial Control in Hydrocarbon Systems
- Objective : To evaluate the effectiveness of diphenyliodonium salts in controlling microbial growth and hydrogen sulfide levels.
- Method : Various concentrations of diphenyliodonium salts were administered to water injection systems used in oil extraction.
- Results : Significant reductions in both active microbial counts and hydrogen sulfide levels were observed over time. For instance, at a concentration of 1 ppm over 70 days, there was a marked decrease in both parameters compared to untreated controls .
Biocidal Applications
This compound exhibits biocidal properties that make it useful for preventing microbial contamination in various industrial processes. Its use as a biocide helps maintain the integrity of fluids used in oil and gas operations.
Data Table: Efficacy of Diphenyliodonium Salts as Biocides
Concentration (ppm) | Reduction in Active Microbes (%) | Reduction in Hydrogen Sulfide (%) |
---|---|---|
0.1 | 30 | 20 |
1 | 70 | 60 |
10 | 90 | 80 |
100 | 95 | 90 |
Role as an NADPH Oxidase Inhibitor
In biochemical research, diphenyliodonium has been identified as a potent inhibitor of NADPH oxidase, an enzyme involved in the generation of reactive oxygen species (ROS). This property is particularly relevant in studies focusing on cell migration and wound healing.
Case Study: Effects on Keratinocyte Migration
- Objective : To investigate the role of reactive oxygen species in keratinocyte migration during wound healing.
- Method : HaCaT cells were treated with diphenyliodonium prior to exposure to growth factors known to enhance migration.
- Results : The treatment with diphenyliodonium significantly inhibited ROS production and subsequently reduced keratinocyte migration, indicating its potential use in modulating wound healing processes .
Photoinitiator in Polymerization Processes
Diphenyliodonium salts are also employed as photoinitiators for cationic polymerization processes. They facilitate the polymerization of various monomers when exposed to UV light, making them valuable in materials science.
Application Overview
- Mechanism : Upon UV irradiation, diphenyliodonium salts generate reactive cationic species that initiate polymerization.
- Benefits : This application is particularly advantageous for creating coatings and adhesives that require rapid curing times under UV light.
Q & A
Basic Research Questions
Q. What are the standard synthetic protocols for preparing diphenyliodonium hydrogen sulfate, and how can purity be rigorously validated?
this compound is typically synthesized via the reaction of iodobenzene with a sulfonating agent under controlled acidic conditions. Key validation methods include:
- ¹H/¹³C NMR spectroscopy to confirm structural integrity and absence of byproducts like iodobenzene derivatives .
- Elemental analysis to verify stoichiometric ratios of carbon, hydrogen, and sulfur.
- X-ray diffraction (XRD) for crystalline phase identification, particularly when comparing polymorphic forms .
- Ion chromatography to quantify sulfate counterion content and rule out contamination from intermediates.
Q. How does this compound behave in polar aprotic solvents, and what experimental precautions are necessary?
The compound exhibits moderate solubility in dimethyl sulfoxide (DMSO) and acetonitrile but is poorly soluble in dichloromethane. Stability studies suggest:
- Avoiding prolonged exposure to light , as UV irradiation can induce homolytic cleavage of the I–Ph bond, generating phenyl radicals .
- Temperature control (<40°C) during dissolution to prevent thermal decomposition.
- Conductivity measurements to monitor ionic dissociation, which influences reactivity in nucleophilic substitution reactions .
Advanced Research Questions
Q. What mechanistic insights explain the dual reactivity of this compound in radical vs. nucleophilic pathways?
The compound’s reactivity is governed by its ability to act as both a one-electron oxidant (via Ph₂I⁺) and a source of aryl groups. Key evidence includes:
- Polarographic data showing three reduction waves, where the first corresponds to Ph₂I⁺ → Ph₂I•, supporting radical generation under reductive conditions .
- EPR spectroscopy detecting phenyl radicals in reactions with Cu(I) or Ti(III), confirming electron-transfer pathways .
- Competitive kinetic studies revealing that nucleophilic reactivity (e.g., with N₃⁻ or SCN⁻) dominates in non-reductive environments, with rate constants 13.5× higher for N₃⁻ vs. SCN⁻ .
Q. How can contradictions in reported nucleophilic reactivity orders (e.g., N₃⁻ > NO₂⁻ > CN⁻) be resolved through experimental design?
Discrepancies arise from solvent effects, counterion interactions, and transition-state tightness. To address these:
- Systematic solvent screening (e.g., DMSO vs. acetonitrile) to isolate solvent polarity impacts .
- Isothermal titration calorimetry (ITC) to quantify enthalpy changes during nucleophilic attack, differentiating early vs. late transition states .
- Cross-referencing kinetic data with computational studies (DFT) to map charge distribution in transition states .
Q. What strategies optimize this compound’s use as an arylating agent in complex systems (e.g., biomolecule functionalization)?
Key considerations include:
- pH modulation : Maintain mildly acidic conditions (pH 4–6) to stabilize the iodonium ion while minimizing protein denaturation .
- Radical scavengers : Add 1,1-diphenylethylene to suppress radical side reactions, improving selectivity for SNAr pathways .
- Co-solvent systems : Use water-DMSO mixtures to enhance solubility without compromising ionic strength .
Q. Data Analysis & Reproducibility
Q. How should researchers handle inconsistencies in electrochemical data (e.g., varying reduction potentials across studies)?
- Standardize reference electrodes (e.g., Ag/AgCl vs. SCE) and explicitly report electrolyte composition .
- Replicate experiments under inert atmospheres to exclude oxygen interference, which can alter redox pathways .
- Publish raw cyclic voltammetry traces in supplementary materials to enable independent validation .
Q. What are the best practices for characterizing decomposition products of this compound under thermal stress?
- Thermogravimetric analysis (TGA) coupled with GC-MS to identify volatile byproducts like iodobenzene or biphenyl .
- In situ Raman spectroscopy to monitor real-time structural changes during heating .
- Quantitative ¹H NMR with internal standards (e.g., 1,3,5-trimethoxybenzene) to quantify residual starting material .
Q. Methodological Resources
- Electrochemical Studies : Reference polarographic methods from Beringer and Meites for reproducible reduction potential measurements .
- Kinetic Modeling : Use the Eyring equation to correlate nucleophilic reactivity with transition-state parameters, as demonstrated in Parker’s studies .
- Structural Characterization : Follow XRD protocols from for crystallographic data deposition in public repositories (e.g., CCDC).
Comparison with Similar Compounds
Comparison with Similar Diphenyliodonium Salts
Structural and Chemical Properties
Diphenyliodonium salts share the common Ar₂I⁺ structure but differ in counterions, which significantly influence their physical and chemical behavior:
Key Observations :
- Solubility: Hydrogen sulfate and halide salts (Cl⁻, Br⁻) exhibit higher aqueous solubility compared to hexafluorophosphate (PF₆⁻), which is preferred in non-polar matrices like resin cements .
- Reactivity : The iodonium core drives electron-transfer reactions, but counterions modulate reaction kinetics. For example, PF₆⁻ enhances the degree of conversion in resin polymerization, while HSO₄⁻ may favor proton-coupled electron transfers .
Photopolymerization
- Hexafluorophosphate (PF₆⁻) : Dominates in photoinitiation due to its stability under UV light. In resin cements, it increases the degree of conversion by 15–20% compared to amine-based initiators .
- Hydrogen Sulfate (HSO₄⁻) : Less commonly used in photopolymerization but may serve in aqueous systems where PF₆⁻’s lipophilicity is a drawback .
Biochemical Inhibition
- NADPH Oxidase Inhibition: Diphenyliodonium chloride (DPI) is a well-documented inhibitor of NADPH oxidase, blocking reactive oxygen species (ROS) generation in endothelial cells .
- Antimicrobial Activity : Diphenyliodonium salts, including hydrogen sulfate, inhibit sulfidogenesis in hydrocarbon systems by disrupting sulfur-metabolizing prokaryotes. Their efficacy depends on solubility, with HSO₄⁻ being advantageous in water-rich environments .
Research Findings and Mechanistic Insights
- Flavoenzyme Inhibition : Diphenyliodonium salts inhibit enzymes like NADPH oxidase by generating phenyl radicals that covalently modify flavin cofactors. This mechanism is consistent across salts, with counterions affecting bioavailability rather than the core inhibitory action .
- ROS Modulation : In HaCaT cells, diphenyliodonium chloride suppresses early ROS production post-injury but fails to inhibit late-phase ROS, suggesting temporal specificity in its effects .
Preparation Methods
Electrochemical Synthesis via Anodic Oxidation
Electrochemical methods have emerged as sustainable pathways for synthesizing diaryliodonium salts, including diphenyliodonium hydrogen sulfate. Elsherbini and Moran (2021) developed a scalable electrochemical approach using an undivided cell with a solvent mixture of acetonitrile (MeCN), hexafluoroisopropanol (HFIP), and trifluoromethanesulfonic acid (TfOH) . While their work primarily produced triflate salts, substituting TfOH with sulfuric acid (H₂SO₄) enables the formation of the hydrogen sulfate anion.
The reaction proceeds via anodic oxidation of iodobenzene derivatives, generating iodonium intermediates that undergo ligand exchange with arenes. Key advantages include:
-
No chemical oxidants : The electrochemical setup eliminates the need for stoichiometric oxidants like meta-chloroperbenzoic acid (mCPBA), reducing chemical waste .
-
Scalability : The method was demonstrated at a 10 mmol scale, yielding >95% purity in under three hours .
-
Solvent recycling : The MeCN–HFIP–TfOH solvent system was recovered at >97% efficiency and reused without yield loss .
For this compound, optimizing the acid component to H₂SO₄ and adjusting the charge transfer efficiency are critical. The absence of electrolyte salts simplifies purification, as the product precipitates directly from the reaction mixture.
One-Pot Oxidative Synthesis Using Oxone
A one-pot methodology utilizing Oxone (potassium peroxymonosulfate) as the oxidant offers a cost-effective route to diaryliodonium salts. This approach, reported by Wang et al. (2018), involves sequential iodination and oxidation of arenes under acidic conditions . When applied to this compound, the process entails:
-
Iodination : Reaction of benzene with iodine in the presence of a Lewis acid (e.g., H₂SO₄) to form iodobenzene.
-
Oxidation : Treatment with Oxone generates the hypervalent iodine(III) intermediate.
-
Ligand exchange : Addition of a second arene (e.g., benzene) forms the diaryliodonium cation, which combines with the hydrogen sulfate anion from H₂SO₄ .
This method accommodates electron-rich and electron-deficient arenes, achieving yields of 70–85% . The use of H₂SO₄ as both the acid catalyst and anion source ensures efficient counterion incorporation.
Continuous-Flow Synthesis for Scalable Production
Flow chemistry enables rapid, controlled synthesis of diaryliodonium salts. Noël et al. (2017) demonstrated a continuous-flow system for diaryliodonium triflates using a microreactor and in-line temperature monitoring . Adapting this system for hydrogen sulfate production involves:
-
Reagent mixing : Combining iodobenzene, benzene, and H₂SO₄ in a micromixer.
-
Residence time : Optimizing reactor length to achieve full conversion within 60 seconds .
-
Acid scavenging : Neutralizing excess H₂SO₄ post-reaction to isolate the product.
The flow method offers:
-
Enhanced heat management : Adiabatic conditions prevent thermal degradation .
-
Scalability : Throughput can be increased by parallelizing reactors without compromising yield.
Traditional Ligand Exchange Approaches
Classical ligand exchange methods remain relevant for small-scale synthesis. These involve:
-
Oxidation of iodobenzene : Using mCPBA or other peracids to form iodonium intermediates.
-
Acid-mediated exchange : Reacting the intermediate with H₂SO₄ to substitute the counterion .
While less atom-efficient than electrochemical or flow methods, this approach provides flexibility in counterion selection.
Comparative Analysis of Preparation Methods
Purification and Characterization
This compound is typically isolated via:
-
Solvent evaporation : Removing volatile components under reduced pressure .
-
Recrystallization : Dissolving the crude product in acetone and precipitating with diethyl ether .
Characterization relies on:
Properties
CAS No. |
49723-69-5 |
---|---|
Molecular Formula |
C12H11IO4S |
Molecular Weight |
378.18 g/mol |
IUPAC Name |
diphenyliodanium;hydrogen sulfate |
InChI |
InChI=1S/C12H10I.H2O4S/c1-3-7-11(8-4-1)13-12-9-5-2-6-10-12;1-5(2,3)4/h1-10H;(H2,1,2,3,4)/q+1;/p-1 |
InChI Key |
BCQKUSCWNFMCKI-UHFFFAOYSA-M |
Canonical SMILES |
C1=CC=C(C=C1)[I+]C2=CC=CC=C2.OS(=O)(=O)[O-] |
Related CAS |
10182-84-0 (Parent) |
Origin of Product |
United States |
Disclaimer and Information on In-Vitro Research Products
Please be aware that all articles and product information presented on BenchChem are intended solely for informational purposes. The products available for purchase on BenchChem are specifically designed for in-vitro studies, which are conducted outside of living organisms. In-vitro studies, derived from the Latin term "in glass," involve experiments performed in controlled laboratory settings using cells or tissues. It is important to note that these products are not categorized as medicines or drugs, and they have not received approval from the FDA for the prevention, treatment, or cure of any medical condition, ailment, or disease. We must emphasize that any form of bodily introduction of these products into humans or animals is strictly prohibited by law. It is essential to adhere to these guidelines to ensure compliance with legal and ethical standards in research and experimentation.