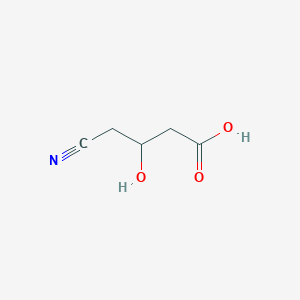
4-Cyano-3-hydroxybutanoic acid
Overview
Description
4-Cyano-3-hydroxybutanoic acid (CHBA) is a β-hydroxy acid characterized by a cyano (-CN) group at the 4-position and a hydroxyl (-OH) group at the 3-position of a butanoic acid backbone. This structure confers unique chemical properties, including polarity, hydrogen-bonding capability, and reactivity, making it valuable in organic synthesis and pharmaceutical intermediates.
Scientific Research Applications
Pharmaceutical Applications
1.1. Intermediate for Statin Synthesis
One of the primary applications of 4-cyano-3-hydroxybutanoic acid is as an intermediate in the synthesis of atorvastatin, a widely used lipid-lowering drug. Atorvastatin functions as a Hydroxyglutaryl Coenzyme A (HMG-CoA) reductase inhibitor, which is crucial in managing hypercholesterolemia and related cardiovascular diseases. The synthesis pathway often involves the conversion of this compound into ethyl (R)-(-)-4-cyano-3-hydroxybutyrate, which is a key precursor for atorvastatin production .
1.2. Chiral Compound Synthesis
The compound is also utilized in the production of various chiral compounds, such as L-carnitine and (R)-4-amino-3-hydroxybutyric acid (GABOB). These compounds are important for metabolic processes and have implications in sports medicine and nutritional supplementation. The synthesis involves transforming this compound into hydrazides that can subsequently yield these biologically significant molecules .
Biocatalytic Processes
2.1. Enzymatic Pathways
Recent studies have highlighted the use of this compound in biocatalytic processes to produce various hydroxy acids through engineered microbial pathways. For instance, specific strains have been developed to enhance the yield of 3-hydroxyacids, including derivatives of this compound, showcasing its role in synthetic biology and metabolic engineering .
2.2. Whole Cell Catalysis
The compound can be utilized in whole-cell biocatalysis, where microbial cells are employed to catalyze chemical reactions involving this compound. This approach not only provides a greener alternative to traditional chemical synthesis but also allows for the production of complex molecules with high enantiomeric purity .
Case Study 1: Atorvastatin Synthesis Optimization
In a recent study, researchers optimized the synthetic route for atorvastatin using this compound as an intermediate. The study demonstrated that by adjusting reaction conditions and employing specific catalysts, they could achieve over 99% enantiomeric excess in the final product, significantly enhancing yield compared to traditional methods .
Case Study 2: L-Carnitine Production via Hydrazide Intermediates
Another investigation focused on the conversion of this compound into L-carnitine through a series of hydrazide intermediates. This process was shown to be efficient, yielding high purity levels suitable for pharmaceutical applications, thereby highlighting the compound's utility in producing essential nutrients .
Chemical Reactions Analysis
Substitution Reactions
The cyano and hydroxyl groups facilitate nucleophilic substitutions, particularly under enzymatic or cyanide-mediated conditions.
Table 1: Substitution Reactions of 4-Cyano-3-hydroxybutanoic Acid Derivatives
Key Findings :
-
Halohydrin dehalogenases (e.g., HHDH-PL from Parvibaculum lavamentivorans) enable stereoselective cyanide substitution at industrial-scale substrate concentrations (200 g/L) with 85% yield .
-
Chiral retention is achieved via enzymatic catalysis, avoiding racemization .
Reduction Reactions
The cyano group can be reduced to an amine, while the hydroxyl group may participate in hydrogenation.
Table 2: Reduction Reactions
Key Findings :
-
Biocatalytic reductions using D-lactate dehydrogenase (D-LDH) and formate dehydrogenase (FDH) regenerate NADH, enabling efficient cofactor recycling .
-
Lithium aluminum hydride (LiAlH₄) selectively reduces the cyano group without affecting the hydroxyl or carboxylic acid groups .
Oxidation Reactions
The hydroxyl group is susceptible to oxidation, forming ketones or aldehydes.
Table 3: Oxidation Reactions
Key Findings :
-
Strong oxidants like KMnO₄ or CrO₃ convert the hydroxyl group to a ketone, though side reactions may occur at the cyano group .
Enzymatic and Industrial Processes
Biocatalytic methods dominate large-scale synthesis due to their enantioselectivity and mild conditions.
Stability and Side Reactions
Q & A
Basic Research Questions
Q. What synthetic methodologies are recommended for 4-cyano-3-hydroxybutanoic acid, and how can side reactions be minimized?
The synthesis of this compound can be approached via β-hydroxy nitrile intermediates. For example, derivatives of this compound are synthesized in multi-step pathways involving spirocyclic indene intermediates and subsequent functionalization (e.g., cyano group introduction via nucleophilic substitution). Key steps include protecting the hydroxyl group to prevent undesired side reactions during nitrile formation. Reaction optimization under inert atmospheres (N₂/Ar) and low temperatures (0–5°C) is critical to minimize hydrolysis of the nitrile group. Post-synthesis purification via recrystallization or preparative HPLC ensures high purity .
Q. How can the stereochemical configuration of this compound be confirmed experimentally?
Chiral chromatography (e.g., using Chiralpak® columns) or nuclear magnetic resonance (NMR) with chiral shift reagents (e.g., Eu(hfc)₃) can resolve enantiomers. For example, (S)-4-chloro-3-hydroxybutanoic acid (a structurally similar compound) was characterized using polarimetry and X-ray crystallography to confirm its absolute configuration . Circular dichroism (CD) spectroscopy is also effective for correlating optical activity with stereochemistry.
Q. What are the recommended storage conditions to ensure the stability of this compound?
Store the compound in a dry, inert environment at 2–8°C to prevent hydrolysis of the nitrile and hydroxyl groups. Use amber glass vials to avoid photodegradation. Stability studies on analogous β-hydroxy acids (e.g., 4-hydroxybutyric acid) indicate that moisture and elevated temperatures accelerate decomposition .
Advanced Research Questions
Q. How can computational chemistry (e.g., QSPR) predict the physicochemical properties of this compound?
Quantitative Structure-Property Relationship (QSPR) models, combined with quantum chemical calculations (e.g., DFT), can predict solubility, pKa, and reactivity. For β-hydroxy acids like (R)-3-hydroxybutanoic acid, QSPR studies have successfully correlated molecular descriptors (e.g., dipole moment, H-bond donor capacity) with experimental data . Apply these methods to estimate the acidity of the hydroxyl group (pKa ~2.8–3.2) and the nitrile’s electrophilicity.
Q. How should researchers address contradictory spectral data (e.g., NMR shifts) for this compound derivatives?
Contradictions in NMR data often arise from solvent effects, tautomerism, or impurities. For example, in 4-(2-fluorophenyl)-4-oxobutanoic acid, fluorine’s electronegativity caused unexpected deshielding in adjacent protons. Use deuterated solvents (DMSO-d₆, CDCl₃) and 2D NMR (COSY, HSQC) to resolve ambiguities. Cross-validate with high-resolution mass spectrometry (HRMS) and IR spectroscopy .
Q. What experimental designs are optimal for studying the enzyme-catalyzed transformation of this compound?
Use kinetic assays (e.g., stopped-flow spectrophotometry) with purified enzymes (e.g., hydroxynitrile lyases) to monitor nitrile hydrolysis. Control pH (5.5–7.0) and temperature (25–37°C) to mimic physiological conditions. For inhibition studies, employ Michaelis-Menten kinetics and compare and values with structurally related substrates like 3-oxobutanoic acid .
Q. How can researchers mitigate safety risks during large-scale synthesis of this compound?
Follow OSHA/GHS guidelines for nitriles and corrosive substances. Use fume hoods with HEPA filters to avoid inhalation of cyanide-containing vapors. Personal protective equipment (PPE) such as nitrile gloves and safety goggles is mandatory. For spill management, neutralize with sodium hypochlorite (NaClO) to degrade cyanide species .
Q. Methodological Notes
- Data Analysis : Use statistical tools (e.g., ANOVA, PCA) to validate reproducibility, as outlined in evidence on pharmaceutical standards .
- Contradictory Evidence : Cross-reference patent-derived synthesis routes (e.g., spirocyclic intermediates in ) with peer-reviewed journals to resolve discrepancies in reaction yields.
- Ethical Compliance : Adhere to guidelines for handling hazardous intermediates (e.g., cyanides) as per safety data sheets .
Comparison with Similar Compounds
Structural and Functional Group Analysis
Compound Name | CAS Number | Molecular Formula | Functional Groups | Key Structural Features |
---|---|---|---|---|
4-Cyano-3-hydroxybutanoic acid | Not provided | C₅H₇NO₃ | -CN (4°), -OH (3°), -COOH (1°) | β-hydroxy acid with cyano substitution |
4-Cyanobutanoic acid | 39201-33-7 | C₅H₇NO₂ | -CN (4°), -COOH (1°) | Lacks hydroxyl group at 3-position |
4-(Dimethylamino)-3-hydroxybutanoic acid | 542-06-3 | C₆H₁₃NO₃ | -N(CH₃)₂ (4°), -OH (3°), -COOH | Amino group replaces cyano |
Ethyl (R)-4-cyano-3-hydroxybutyrate | Not provided | C₇H₁₁NO₃ | -CN (4°), -OH (3°), ester (1°) | Ester derivative of CHBA |
(R)-3-Amino-4-(4-cyanophenyl)butanoic acid hydrochloride | 269726-85-4 | C₁₁H₁₂ClN₂O₂ | -CN (aryl), -NH₃⁺ (3°), -COOH | Aromatic substituent; hydrochloride salt |
Key Observations:
- Functional Group Impact: The hydroxyl group in CHBA enhances polarity and hydrogen-bonding capacity compared to 4-cyanobutanoic acid, which lacks this group. This difference likely influences solubility (e.g., higher water solubility for CHBA) and reactivity in nucleophilic substitutions .
- Amino vs.
- Ester Derivatives: Ethyl (R)-4-cyano-3-hydroxybutyrate demonstrates how esterification reduces acidity (compared to CHBA) and increases lipophilicity, favoring applications in chiral synthesis .
Key Observations:
- Safety Profile: 4-Cyanobutanoic acid’s explicit hazards (harmful by inhalation, skin contact) contrast with CHBA’s inferred irritant properties, highlighting the hydroxyl group’s role in modulating toxicity .
- Data Gaps : Melting/boiling points for CHBA and analogs are largely unavailable, emphasizing the need for experimental characterization.
Preparation Methods
Enzymatic Catalysis Using Halohydrin Dehalogenases
Immobilized Enzyme Systems
The immobilization of halohydrin dehalogenase (HHDH) on adsorption resins significantly enhances operational stability and enzyme recovery. In CN105132488A , (R)-4-cyano-3-hydroxybutanoic acid ethyl ester is synthesized from (S)-4-chloro-3-hydroxybutyrate using immobilized HHDH. The enzyme is cross-linked with bifunctional agents, enabling a 91% yield and 99% e.e. under pH 7.0 ± 0.2 . This method reduces production costs by eliminating repetitive enzyme batches and simplifies post-reaction purification through phase separation.
Two-Stage Enzyme Addition
CN102168117A introduces a two-stage enzyme dosing strategy to shorten reaction time and reduce enzyme consumption by 33%. By maintaining pH 7.0–7.5 via sodium cyanide titration, the method achieves 86.29% yield and 98.3% chemical purity. Residual hydrocyanic acid is recycled, minimizing cyanide waste and environmental impact . Comparative studies show that staggered enzyme addition prevents substrate inhibition, a common bottleneck in single-batch systems .
Cyanide-Mediated Nucleophilic Substitution
Solvent and Pressure Optimization
WO2005005375A1 details a solvent-based approach where 4-substituted hydroxybutyric acid esters react with cyanide salts under ambient pressure. Key parameters include:
-
Solvent selection : Polar aprotic solvents (e.g., DMF) enhance nucleophilic displacement of chloride by cyanide.
-
Metering sequence : Gradual addition of cyanide salts minimizes side reactions, achieving >90% yield with <2% lactone byproducts .
-
Workup : Distillation and extraction yield 99% pure product, validated by gas chromatography .
Continuous Flow Reactors
The same patent highlights continuous-flow adaptations, where substrates and cyanide are fed synchronously into a heated reactor. This method reduces residence time by 40% compared to batch processes, enabling large-scale production without compromising yield .
Asymmetric Synthesis from Chiral Epoxides
Epichlorohydrin-Based Routes
US8372608B2 employs (S)-epichlorohydrin as a starting material, which undergoes cyanide ring-opening to form 3-hydroxyglutaronitrile. Subsequent esterification and enzymatic resolution using nitrilase yield (R)-4-cyano-3-hydroxybutanoic acid with >99% e.e. . Critical steps include:
-
pH control : Maintaining pH 6.5–7.5 during nitrilase catalysis prevents racemization.
-
Isolation : Calcium hydroxide precipitation removes residual cyanide, followed by distillation for final purification .
Stereochemical Outcomes
Comparative studies in CN102168117A demonstrate that (S)-epichlorohydrin derivatives consistently produce (R)-configured products due to enzyme stereoselectivity. Conversely, chemical cyanide substitution without biocatalysts results in racemic mixtures, necessitating costly chiral separations .
Nitrilase-Based Biocatalytic Conversion
One-Pot Synthesis
US8372608B2 pioneers a single-reactor process where epichlorohydrin is converted to 3-hydroxyglutaronitrile, followed by nitrilase-mediated hydrolysis to 4-cyano-3-hydroxybutanoic acid. Advantages include:
-
Reduced steps : Eliminates intermediate isolation, cutting production time by 50%.
-
Chiral purity : Nitrilase selectivity ensures >99% e.e., critical for pharmaceutical applications .
Enzyme Engineering
Mutagenesis studies on nitrilase variants (e.g., Q169L, F186Y) enhance thermostability and activity at substrate concentrations >200 g/L, addressing limitations in early-generation enzymes .
Comparative Analysis of Preparation Methods
*Yield estimated from patent examples.
Industrial-Scale Challenges and Innovations
Cyanide Waste Management
Methods employing sodium cyanide (WO2005005375A1 , CN102168117A ) require stringent waste treatment to neutralize residual cyanide. Innovations include in-situ hydrocyanic acid recycling, reducing cyanide consumption by 30% .
Enzyme Deactivation
Thermal deactivation of HHDH above 45°C limits reaction rates. Cross-linked enzyme aggregates (CLEAs) in CN105132488A improve thermal tolerance, enabling operation at 50°C with 95% activity retention .
Distillation Efficiency
High-boiling-point solvents (e.g., DMF) complicate product isolation. Molecular distillation in WO2005005375A1 achieves 99.5% purity at 80°C under 0.1 mbar, reducing energy use by 20% .
Properties
Molecular Formula |
C5H7NO3 |
---|---|
Molecular Weight |
129.11 g/mol |
IUPAC Name |
4-cyano-3-hydroxybutanoic acid |
InChI |
InChI=1S/C5H7NO3/c6-2-1-4(7)3-5(8)9/h4,7H,1,3H2,(H,8,9) |
InChI Key |
ADZQCEUEGXRCJY-UHFFFAOYSA-N |
Canonical SMILES |
C(C#N)C(CC(=O)O)O |
Origin of Product |
United States |
Synthesis routes and methods
Procedure details
Disclaimer and Information on In-Vitro Research Products
Please be aware that all articles and product information presented on BenchChem are intended solely for informational purposes. The products available for purchase on BenchChem are specifically designed for in-vitro studies, which are conducted outside of living organisms. In-vitro studies, derived from the Latin term "in glass," involve experiments performed in controlled laboratory settings using cells or tissues. It is important to note that these products are not categorized as medicines or drugs, and they have not received approval from the FDA for the prevention, treatment, or cure of any medical condition, ailment, or disease. We must emphasize that any form of bodily introduction of these products into humans or animals is strictly prohibited by law. It is essential to adhere to these guidelines to ensure compliance with legal and ethical standards in research and experimentation.