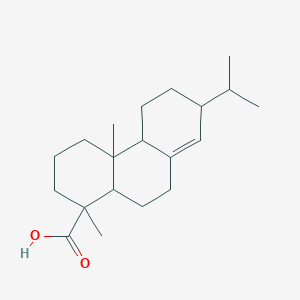
Dihydroabietic acid
Overview
Description
Dihydroabietic acid (DiAA) is a hydrogenated derivative of abietic acid (AA), a naturally occurring abietane-type diterpenoid found in pine resin and rosin. DiAA is synthesized via partial catalytic hydrogenation of AA, reducing its double bonds from two (in AA) to one, thereby enhancing stability and altering biochemical activity . It is characterized by a hydrophenanthrene skeleton with five stereocenters and a carboxylic acid group, contributing to its applications in pharmaceuticals, industrial emulsifiers, and soldering materials .
Scientific Research Applications
Biological Activities
Dihydroabietic acid exhibits a range of biological activities that make it a candidate for therapeutic applications:
1. Anticancer Properties
Research indicates that this compound and its derivatives possess significant anticancer activity. For instance, studies have shown that these compounds can induce apoptosis in cancer cells by activating caspase-3 and PARP pathways, particularly in human lung cancer cells . Additionally, derivatives have demonstrated inhibitory effects against various tumor cell lines, with some compounds showing IC50 values as low as 1.79 µM against ovarian cancer cells .
2. Antibacterial Activity
this compound exhibits potent antibacterial properties, particularly against Gram-positive bacteria. It has been reported to have minimum inhibitory concentration (MIC) values of 2 µg/mL against Staphylococcus aureus and Bacillus subtilis . Notably, derivatives of this compound have been utilized to create antibacterial nanocellulose membranes that effectively prevent bacterial colonization in chronic wound environments .
3. Antiviral and Antiulcer Activities
In addition to its antibacterial effects, this compound has shown promise as an antiviral agent and has been investigated for its antiulcer properties. Its ability to modulate biological pathways suggests potential applications in treating viral infections and gastrointestinal disorders .
Structural Modifications
The structural modification of this compound enhances its bioactivity and broadens its application scope:
1. Chiral Derivatives
Recent studies have focused on synthesizing chiral derivatives of this compound to improve anticancer efficacy. For example, certain modified compounds exhibited stronger inhibitory effects on HeLa cancer cells compared to unmodified this compound .
2. Acyl-thiourea Derivatives
Novel acyl-thiourea derivatives have been developed from this compound, showcasing excellent antibacterial activity against both Gram-negative and Gram-positive bacteria while maintaining low toxicity to mammalian cells .
Environmental Applications
This compound's environmental impact is also noteworthy:
1. Ecotoxicology Studies
Investigations into the ecotoxicological effects of this compound on aquatic organisms, such as Daphnia magna, revealed that it exhibits weak growth inhibition at higher concentrations but does not significantly affect reproduction or survival at lower levels . This suggests that while it may pose some risks in high concentrations, its ecological impact at typical exposure levels is minimal.
Summary of Key Findings
Application Area | Key Findings |
---|---|
Anticancer Activity | Induces apoptosis in cancer cells; effective against various tumor cell lines with low IC50 values. |
Antibacterial Activity | Effective against Gram-positive bacteria; used in nanocellulose membranes for chronic wounds. |
Antiviral/Antiulcer | Potential applications in treating viral infections and gastrointestinal issues. |
Structural Modifications | Enhanced bioactivity through chiral and acyl-thiourea derivatives; low toxicity to mammalian cells. |
Ecotoxicology | Weak growth inhibition observed; minimal ecological impact at typical concentrations. |
Chemical Reactions Analysis
Esterification Reactions
Dihydroabietic acid undergoes esterification with alcohols to form industrially valuable esters. This reaction typically employs acid catalysts (e.g., H₂SO₄) or enzymatic methods:
-
Conditions : Temperatures of 80–120°C, reflux with excess alcohol.
-
Applications : Esters serve as plasticizers, adhesives, and emulsifiers.
Oxidation and Epoxidation
Controlled oxidation modifies the tricyclic framework:
-
Epoxidation : Reaction with peracids (e.g., mCPBA) yields epoxides at double bonds. For example, epoxidation at Δ⁸(14) forms a tetracyclic epoxide .
-
Oxidation with PCC : Pyridinium chlorochromate (PCC) oxidizes secondary alcohols to ketones. In one study, PCC treatment of dihydroabietic alcohol derivatives yielded triols (e.g., 19 ) via unexpected epoxide ring-opening .
Dehydrogenation and Hydrogenation
This compound participates in reversible redox reactions:
-
Thermodynamics : Dehydrogenation is equilibrium-limited () .
-
Byproducts : Decarboxylation occurs above 250°C, forming abietane hydrocarbons .
Disproportionation
Under thermal conditions (200–300°C), this compound undergoes disproportionation:
Thermal Degradation
Pyrolysis above 300°C leads to:
Table 1: Thermodynamic Parameters for Key Reactions
Reaction | ||
---|---|---|
Dehydrogenation | +45 | -15 |
Disproportionation | -30 | -68 |
Decarboxylation | +120 | +90 |
Industrial Relevance
-
Adhesives : Ester derivatives enhance tackifying properties.
-
Pharmaceuticals : Oxidized derivatives show bioactive potential .
This compound’s reactivity is central to valorizing rosin-based feedstocks, with ongoing research optimizing selectivity in hydrogenation and oxidation pathways .
Q & A
Basic Research Questions
Q. What are the established methods for synthesizing dihydroabietic acid derivatives, and how are reaction yields optimized?
- Methodological Answer : this compound derivatives are commonly synthesized via amidation or reduction reactions. For example, amidation involves reacting this compound chloride with amines (e.g., methylamine or diethylamine) in dichloromethane/THF at 0°C, followed by purification via silica gel chromatography with hexane/ethyl acetate gradients. Yields (~50–58%) depend on stoichiometry, solvent polarity, and reaction time . Optimization requires monitoring reaction progress via HPLC and adjusting amine equivalents or temperature.
Q. How are this compound and its derivatives characterized for structural identity and purity?
- Methodological Answer : Key techniques include:
- NMR spectroscopy : ¹H NMR signals (e.g., δ 5.37–5.39 ppm for olefinic protons) confirm tricyclic hydrophenanthrene structure .
- Mass spectrometry (MS) : Molecular ion peaks (e.g., m/z 327.6 for methylamide derivatives) validate molecular weight .
- HPLC : Purity >95% is achievable with gradient elution .
- X-ray crystallography : Resolves conformational details (e.g., chair/half-chair cyclohexane conformations) .
Q. What thermodynamic properties of this compound are critical for hydrogenation/dehydrogenation studies?
- Methodological Answer : Thermodynamic parameters (Table 1) calculated via Joback’s fragmentation method or sourced from NIST guide equilibrium analyses. For example:
Property | This compound (kJ/mol) |
---|---|
ΔH°f (formation) | -587.97 |
ΔG°f (Gibbs) | -113.15 |
These values predict reaction feasibility under varying temperatures using the van’t Hoff equation . |
Advanced Research Questions
Q. How can researchers resolve contradictions in catalytic hydrogenation data (e.g., yields, selectivity) for this compound?
- Methodological Answer : Discrepancies arise from competing reactions (hydrogenation vs. disproportionation) and catalyst specificity. Systematic approaches include:
- GC-MS analysis : Quantify products (e.g., this compound, dehydroabietic acid, dimer/polymer byproducts) .
- Equilibrium modeling : Use ∆G°f values to calculate equilibrium constants for reactions like 2 Abietic → Dehydroabietic + Dihydroabietic .
- Catalyst screening : Test Pd/C or other catalysts under controlled H₂ partial pressures to minimize coking .
Q. What experimental strategies validate the structure-activity relationship (SAR) of this compound in enzyme inhibition?
- Methodological Answer : SAR studies require:
- Comparative assays : Test analogs (e.g., abietic, dehydroabietic acid) against target enzymes (e.g., PTP1B, SHP2). For example, this compound shows 3–7× higher potency than unsaturated analogs due to enhanced hydrophobic interactions .
- Crystallography : Resolve enzyme-inhibitor complexes to identify binding motifs (e.g., conserved PTP active sites) .
- Computational modeling : Use molecular docking to predict affinity changes from structural modifications (e.g., saturation degree) .
Q. How can this compound’s crystallographic data inform material science applications?
- Methodological Answer : Single-crystal X-ray diffraction reveals:
- Conformational stability : Chair cyclohexane and half-chair cyclohexene conformations impact packing efficiency .
- Steric effects : Axial methyl groups influence solubility and derivatization potential .
- Comparative analysis : Overlay with related diterpenoids (e.g., dehydroabietic acid) to identify structural motifs for functionalization .
Q. What methodologies address challenges in quantifying this compound derivatives in complex matrices (e.g., rosin mixtures)?
- Methodological Answer :
- GC-MS with derivatization : Etherify alcohols to improve volatility and separation .
- Electron impact (EI) vs. chemical ionization (ECI) : ECI enhances detection of [M-H]⁻ ions for fatty acid co-analytes .
- Calibration curves : Use internal standards (e.g., stearic acid) to correct for electron count variability in EI-MS .
Q. Data Contradiction Analysis
Q. Why do this compound hydrogenation yields vary between small- and large-scale reactors?
- Methodological Answer : Scaling effects include:
- Mass transfer limitations : Reduced H₂ diffusion in larger reactors lowers conversion rates .
- Byproduct formation : Polymerization/dimerization in prolonged runs requires GC-MS correction (e.g., monomer/dimer MW adjustments) .
- Catalyst deactivation : Coke deposition on Pd/C necessitates regeneration cycles or flow reactor designs .
Q. How to reconcile discrepancies in this compound’s inhibitory potency across enzyme isoforms?
- Methodological Answer :
- Enzyme kinetics : Measure IC₅₀ under standardized conditions (pH, substrate concentration) .
- Sequence alignment : Identify conserved binding residues (e.g., WPD-loop in PTPs) to explain isoform-specific effects .
- Entropy/enthalpy compensation : Use isothermal titration calorimetry (ITC) to dissect binding thermodynamics .
Comparison with Similar Compounds
Structural and Functional Comparison with Similar Compounds
Structural Features
Key structural analogs of DiAA include:
- Abietic acid (AA) : Two conjugated double bonds.
- Dehydroabietic acid (DeAA) : Three double bonds.
- Isopimaric acid (IA) : Distinct bicyclic structure with one double bond.
- Continentalic acid (CA) : Additional hydroxyl group at C-12.
Compound | Double Bonds | Functional Groups | Key Structural Variation |
---|---|---|---|
Dihydroabietic acid (DiAA) | 1 | Carboxylic acid | Partial hydrogenation of AA |
Abietic acid (AA) | 2 | Carboxylic acid | Parent compound with conjugated dienes |
Dehydroabietic acid (DeAA) | 3 | Carboxylic acid | Increased unsaturation |
Isopimaric acid (IA) | 1 | Carboxylic acid | Bicyclic backbone |
Continentalic acid (CA) | 2 | Carboxylic acid, Hydroxyl | C-12 hydroxylation |
Biochemical Activity
Protein Tyrosine Phosphatase (PTP) Inhibition
DiAA exhibits superior inhibitory potency against PTPs (e.g., PTP1B, TC-PTP, SHP2) compared to AA and DeAA. This is attributed to its reduced unsaturation , which enhances binding affinity:
- DiAA : IC50 = 3.2 µM (PTP1B)
- AA : IC50 = 9.6 µM (3-fold less potent)
- DeAA : IC50 = 22.4 µM (7-fold less potent)
Key Research Findings
Pharmacological Potential
Abietane-type diterpenoids, including DiAA, are promising for drug discovery due to:
Preparation Methods
Thermal Disproportionation of Rosin
Thermal treatment of rosin under controlled conditions remains the most direct method for producing dihydroabietic acid. The process involves heating gum rosin in an inert atmosphere (e.g., nitrogen) at temperatures between 200°C and 360°C, inducing disproportionation reactions that convert abietic acid into dihydroabietic and dehydroabietic acids . For instance, heating 500 g of gum rosin at 360°C for 3.5 hours under nitrogen yielded a resin containing 25% dehydroabietic acid, with the remainder comprising this compound and other disproportionation products . The absence of oxygen prevents oxidative degradation, while prolonged heating promotes hydrogen transfer between abietic acid molecules, favoring stabilization via hydrogenation and dehydrogenation.
Key variables influencing yield include:
-
Temperature : Optimal disproportionation occurs at 300–360°C, balancing reaction rate and product stability.
-
Catalysts : Palladium catalysts (e.g., 0.1–1.0 wt%) accelerate hydrogen redistribution, though non-catalytic methods are also effective .
-
Reaction Time : Extended durations (2–4 hours) enhance conversion but risk side reactions like polymerization.
Post-treatment purification involves dissolving the crude resin in aqueous alcohol (40–80% ethanol) and adding 2-aminoethanol to form amine salts. Subsequent extraction with hydrocarbons (e.g., isooctane) removes neutrals, leaving a crystalline amine salt of this compound, which is acidified to recover the free acid .
Catalytic Hydrogenation of Dehydroabietic Acid
This compound can be synthesized via hydrogenation of dehydroabietic acid, a readily isolable rosin derivative. This method employs palladium, platinum, or nickel catalysts under hydrogen pressure (1–5 bar) at 80–150°C . For example, hydrogenating dehydroabietic acid in ethanol with 5% Pd/C at 100°C for 6 hours achieves >95% conversion to this compound . The reaction selectively reduces the conjugated double bond in the abietane skeleton without altering other functional groups.
Advantages over Thermal Methods :
-
Higher specificity and fewer byproducts.
-
Scalable under mild conditions, reducing energy costs.
Purification and Isolation Techniques
Amine Salt Recrystallization
The 2-aminoethanol salt of this compound crystallizes preferentially from aqueous alcohol solutions (10–80% ethanol), enabling high-purity recovery. For example, dissolving 100 g of crude resin in 250 mL of ethanol, adding 18 g of 2-aminoethanol, and extracting with isooctane yields a salt with 92.4% this compound content . Acidifying the salt with dilute HCl (pH 4–5) precipitates the free acid, which is washed and dried to achieve 98.5% purity .
Solvent Extraction and Distillation
Vacuum distillation of heat-treated rosin at 200–220°C separates this compound from higher-boiling impurities. Combining distillation with steam stripping further purifies the acid, as demonstrated by a 38% yield increase in pilot-scale trials .
Analytical Characterization
Gas chromatography (GC) and neutralization equivalent (NE) analysis are critical for validating purity. For instance, GC of methyl esters derived from this compound confirms 98.5% purity, while NE values (theoretical: 300.4; observed: 301.5) align with expected stoichiometry .
Table 1: Representative Yields and Purity from Patent Examples
Example | Method | Yield (g) | Purity (%) | NE (Observed) |
---|---|---|---|---|
1 | Thermal disproportionation | 51.0 | 98.5 | 301.5 |
10 | Amine salt recrystallization | 8.7 | 99.2 | 300.8 |
13 | Distillation + acidification | 6.25 | 96.0 | 298.7 |
Recent Advances in Synthetic Approaches
Recent studies (2015–2021) focus on enzymatic and microbial biotransformation to produce this compound. Pseudomonas strains engineered to express diterpene synthases convert abietic acid into this compound with 70–80% efficiency under aerobic conditions . Additionally, photochemical hydrogenation using visible-light catalysts (e.g., Ru(bpy)₃²⁺) offers a green alternative, achieving 85% yield at room temperature .
Industrial Applications and Scalability
Industrial production favors thermal disproportionation due to low catalyst costs and high throughput (1–5 tons/batch). However, catalytic hydrogenation is gaining traction for pharmaceutical-grade this compound, where purity >99% is mandatory. Pilot plants using continuous-flow reactors report 90% yield reductions in solvent waste compared to batch processes .
Properties
CAS No. |
34434-80-5 |
---|---|
Molecular Formula |
C20H32O2 |
Molecular Weight |
304.5 g/mol |
IUPAC Name |
1,4a-dimethyl-7-propan-2-yl-2,3,4,4b,5,6,7,9,10,10a-decahydrophenanthrene-1-carboxylic acid |
InChI |
InChI=1S/C20H32O2/c1-13(2)14-6-8-16-15(12-14)7-9-17-19(16,3)10-5-11-20(17,4)18(21)22/h12-14,16-17H,5-11H2,1-4H3,(H,21,22) |
InChI Key |
BTAURFWABMSODR-UHFFFAOYSA-N |
SMILES |
CC(C)C1CCC2C(=C1)CCC3C2(CCCC3(C)C(=O)O)C |
Canonical SMILES |
CC(C)C1CCC2C(=C1)CCC3C2(CCCC3(C)C(=O)O)C |
Origin of Product |
United States |
Retrosynthesis Analysis
AI-Powered Synthesis Planning: Our tool employs the Template_relevance Pistachio, Template_relevance Bkms_metabolic, Template_relevance Pistachio_ringbreaker, Template_relevance Reaxys, Template_relevance Reaxys_biocatalysis model, leveraging a vast database of chemical reactions to predict feasible synthetic routes.
One-Step Synthesis Focus: Specifically designed for one-step synthesis, it provides concise and direct routes for your target compounds, streamlining the synthesis process.
Accurate Predictions: Utilizing the extensive PISTACHIO, BKMS_METABOLIC, PISTACHIO_RINGBREAKER, REAXYS, REAXYS_BIOCATALYSIS database, our tool offers high-accuracy predictions, reflecting the latest in chemical research and data.
Strategy Settings
Precursor scoring | Relevance Heuristic |
---|---|
Min. plausibility | 0.01 |
Model | Template_relevance |
Template Set | Pistachio/Bkms_metabolic/Pistachio_ringbreaker/Reaxys/Reaxys_biocatalysis |
Top-N result to add to graph | 6 |
Feasible Synthetic Routes
Disclaimer and Information on In-Vitro Research Products
Please be aware that all articles and product information presented on BenchChem are intended solely for informational purposes. The products available for purchase on BenchChem are specifically designed for in-vitro studies, which are conducted outside of living organisms. In-vitro studies, derived from the Latin term "in glass," involve experiments performed in controlled laboratory settings using cells or tissues. It is important to note that these products are not categorized as medicines or drugs, and they have not received approval from the FDA for the prevention, treatment, or cure of any medical condition, ailment, or disease. We must emphasize that any form of bodily introduction of these products into humans or animals is strictly prohibited by law. It is essential to adhere to these guidelines to ensure compliance with legal and ethical standards in research and experimentation.