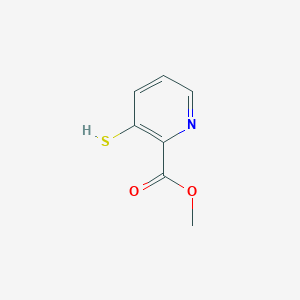
Methyl 3-mercaptopicolinate
- Click on QUICK INQUIRY to receive a quote from our team of experts.
- With the quality product at a COMPETITIVE price, you can focus more on your research.
Overview
Description
3-Mercaptopicolinic acid (3-MP), a thiol-containing derivative of picolinic acid, is a well-characterized inhibitor of phosphoenolpyruvate carboxykinase (PEPCK), a critical enzyme in gluconeogenesis. It reversibly binds to the active site of PEPCK, disrupting the conversion of oxaloacetate to phosphoenolpyruvate (PEP), thereby reducing glucose synthesis . Key findings include:
- Mechanism: 3-MP acts as a noncompetitive inhibitor with respect to oxaloacetate and Mn²⁺-GTP, with inhibition constants (Ki) ranging from 3 to 9 μM. It induces conformational changes in PEPCK without forming mixed disulfide bonds or chelating Mn²⁺ .
- Reversibility: Inhibition is fully reversible, as demonstrated by activity restoration after 3-MP removal via desalting columns .
- Species-Specific Effects: In guinea pig liver, 3-MP (100 μM) completely blocks gluconeogenesis from glycerol, whereas in rat liver, inhibition is partial .
Scientific Research Applications
Biochemical Mechanisms and Metabolic Regulation
Methyl 3-mercaptopicolinate, a derivative of 3-mercaptopicolinic acid, has been shown to significantly inhibit gluconeogenesis, particularly in liver tissues. Research indicates that concentrations of this compound can lead to a marked decrease in glucose synthesis from lactate and glycerol in isolated liver models. Specifically, at a concentration of 100 µM, it completely inhibits gluconeogenesis from lactate in guinea pig livers, while showing partial inhibition in rat livers .
Table 1: Inhibition of Gluconeogenesis by this compound
Concentration (µM) | Effect on Gluconeogenesis |
---|---|
50 | Sharp decrease |
100 | Complete inhibition |
The mechanism of action involves noncompetitive inhibition of phosphoenolpyruvate carboxykinase, a key enzyme in the gluconeogenic pathway. This inhibition is primarily due to decreased product formation rather than alterations in substrate affinity .
Diabetes Management
Given its ability to inhibit gluconeogenesis, this compound has potential applications in managing hyperglycemia and diabetes. Studies have demonstrated that treatment with this compound can enhance insulin sensitivity while reducing hepatic glucose production . This dual action could make it a candidate for further investigation as a therapeutic agent for type 2 diabetes.
Neurological Disorders
Recent research has explored the effects of this compound in models of epilepsy. In particular, the compound has been associated with modulation of metabolic pathways that are disrupted in seizure disorders. Its role in enhancing glucose metabolism may contribute to neuroprotective effects, warranting further exploration in the context of epilepsy treatment .
Enzyme Inhibition Studies
This compound has been investigated for its potential as an enzyme inhibitor. Specifically, it has shown promise in inhibiting phosphoenolpyruvate carboxykinase activity, which is crucial for gluconeogenesis and other metabolic processes .
Table 2: Enzyme Inhibition Profile
Enzyme | Inhibition Type | Concentration (µM) |
---|---|---|
Phosphoenolpyruvate Carboxykinase | Noncompetitive | 50 - 100 |
The implications of this inhibition extend beyond metabolic regulation; they suggest potential applications in cancer therapy where metabolic reprogramming is a strategy for targeting tumor growth .
Case Studies and Research Findings
Several studies have documented the effects of this compound on various biological systems:
- Diabetes Research : A study demonstrated that treatment with this compound significantly reduced endogenous glucose production in mice. This was evidenced by decreased glucose levels following pyruvate tolerance tests .
- Epilepsy Models : Investigations using animal models have shown that this compound can modulate metabolic pathways affected by seizures, potentially offering a new avenue for therapeutic intervention .
Q & A
Basic Research Questions
Q. What are the established synthetic routes for Methyl 3-mercaptopicolinate, and how can their efficiencies be systematically compared?
- Methodological Answer : Compare synthetic pathways (e.g., nucleophilic substitution, thiolation of picolinate derivatives) by evaluating reaction yields, purity (via HPLC or GC-MS), and scalability. Use kinetic studies (e.g., time-resolved NMR) to monitor intermediate formation and optimize reaction conditions (temperature, solvent polarity, catalyst loading). Include control experiments to rule out side reactions, and validate purity through elemental analysis and spectroscopic characterization (¹H/¹³C NMR, IR) .
Q. Which analytical techniques are most reliable for characterizing the structural integrity and purity of this compound?
- Methodological Answer : Prioritize hyphenated techniques such as LC-MS for purity assessment, coupled with ¹H/¹³C NMR for structural confirmation. For quantitative purity, use HPLC with a calibrated standard. Differential Scanning Calorimetry (DSC) can determine thermal stability, while X-ray crystallography provides definitive proof of molecular geometry. Report detection limits and reproducibility metrics (e.g., %RSD for triplicate analyses) to ensure data robustness .
Q. How does solvent choice impact the stability of this compound during storage and reactions?
- Methodological Answer : Conduct accelerated stability studies in polar (e.g., DMSO, methanol) and nonpolar solvents (e.g., hexane) under varying temperatures. Monitor degradation via UV-Vis spectroscopy or LC-MS at fixed intervals. Use Arrhenius modeling to predict shelf-life and identify degradation byproducts. Include controls with antioxidants (e.g., BHT) to assess protective effects .
Advanced Research Questions
Q. What mechanistic insights explain the regioselective reactivity of this compound in cross-coupling reactions?
- Methodological Answer : Employ density functional theory (DFT) calculations to map potential energy surfaces for reaction intermediates. Validate computational models with experimental kinetic isotope effects (KIE) or Hammett plots. Use in-situ FTIR or Raman spectroscopy to detect transient species. Compare results with analogous compounds (e.g., methyl 2-mercaptopicolinate) to isolate electronic vs. steric influences .
Q. How can contradictions in reported biological activity data for this compound be resolved?
- Methodological Answer : Perform meta-analyses of existing datasets to identify confounding variables (e.g., cell line variability, assay protocols). Replicate key studies under standardized conditions (e.g., ISO 17025 guidelines) with positive/negative controls. Use multivariate statistics (e.g., PCA) to isolate factors contributing to discrepancies. Publish raw data in open-access repositories to enable third-party validation .
Q. What strategies optimize the enantiomeric resolution of this compound derivatives for chiral catalysis applications?
- Methodological Answer : Screen chiral stationary phases (CSPs) via HPLC or SFC with polysaccharide-based columns (e.g., Chiralpak AD-H). Vary mobile phase composition (e.g., hexane:isopropanol ratios) to achieve baseline separation. Correlate enantiomeric excess (ee) with catalytic performance using kinetic resolution experiments. Report resolution factors (Rs) and asymmetry indices for transparency .
Q. How does this compound interact with transition-metal catalysts in C–S bond-forming reactions?
- Methodological Answer : Use X-ray absorption spectroscopy (XAS) to probe metal-ligand coordination geometry. Perform stoichiometric reactions with isolated metal complexes (e.g., Pd(0)/Pd(II)) and analyze intermediates via ESI-MS. Compare turnover frequencies (TOF) under inert vs. aerobic conditions to assess catalyst stability. Cross-reference with computational studies to validate proposed mechanisms .
Q. Data Presentation and Reproducibility Guidelines
- Tables : Include comparative data (e.g., reaction yields, spectroscopic peaks) with error margins (±SD) and statistical significance (p-values) where applicable .
- Figures : Use high-resolution schematics for reaction mechanisms and avoid overcrowding with chemical structures (limit to 2–3 per graphic) .
- Ethical Reporting : Disclose conflicts of interest, adhere to IUPAC nomenclature, and provide raw data in supplementary materials .
Comparison with Similar Compounds
Quinolinate
- Inhibition Mechanism: Quinolinate, like 3-MP, inhibits PEPCK but exhibits lower potency. It primarily affects mitochondrial PEPCK isoforms, while 3-MP targets both cytosolic and mitochondrial forms .
3-Aminopicolinate
- Metal Interactions: The Fe²⁺ complex of 3-aminopicolinate activates PEPCK, whereas the Fe²⁺-3-MP complex inhibits it. This highlights divergent roles of metal coordination in modulating enzyme activity .
EDTA and Sulfhydryl Reagents
- Non-Specific Inhibition: EDTA (a metal chelator) and sulfhydryl reagents (e.g., N-ethylmaleimide) inhibit PEPCK non-specifically by disrupting metal cofactors or forming disulfide bonds. In contrast, 3-MP’s inhibition is specific and reversible .
Methyl 2-Methyl-3-Mercaptopropionate
- Structural Differences: This aliphatic mercapto compound (CAS 4131-76-4) lacks the pyridine ring of 3-MP, resulting in distinct biochemical properties.
Data Tables
Table 1: Inhibition Constants (Ki) of PEPCK Inhibitors
Table 2: Species-Specific Effects of 3-MP on Gluconeogenesis
Table 3: Binding Mechanisms and Conformational Effects
Key Research Findings and Contradictions
- Mn²⁺ Binding : Early studies suggested 3-MP inhibits PEPCK by displacing Mn²⁺, but later work showed the Mn²⁺-3-MP complex has a dissociation constant of 0.51 mM, ruling out direct metal chelation .
- Quaternary Complex Formation : High-resolution ^1H NMR confirmed the formation of E·Mn·3-MP·PEP complexes, though PRR titration initially failed to detect them .
- Hormonal Interactions : In hepatocytes, 3-MP decreases fatty acid esterification, but catecholamines counteract this effect, suggesting interplay between PEPCK inhibition and hormonal regulation .
Preparation Methods
Direct Esterification via Acid-Catalyzed Reaction
The most widely documented method for synthesizing methyl 3-mercaptopicolinate involves the direct esterification of 3-mercaptopicolinic acid with methanol. This reaction follows the Fisher esterification mechanism, where a carboxylic acid reacts with an alcohol in the presence of an acid catalyst to yield an ester and water .
Reaction Mechanism and Conditions
The reaction proceeds via protonation of the carboxylic acid group, followed by nucleophilic attack by methanol to form a tetrahedral intermediate. Subsequent dehydration yields the ester. Key parameters include:
-
Catalyst : Concentrated sulfuric acid (H2SO4) serves dual roles as a catalyst and dehydrating agent, shifting the equilibrium toward ester formation by removing water .
-
Temperature : Reflux conditions (140–180°C) are employed to maintain reaction kinetics while preventing methanol evaporation .
-
Molar Ratios : A molar excess of methanol (typically 4:1 alcohol-to-acid ratio) ensures maximal conversion of the carboxylic acid .
Purification and Yield Optimization
Post-reaction purification involves neutralizing excess acid, followed by solvent extraction and recrystallization. Industrial-scale processes often incorporate vacuum distillation to isolate the ester . Yields are highly dependent on reaction duration and water removal efficiency, with laboratory reports indicating 60–75% efficiency under optimized conditions .
Industrial Synthesis and Process Scalability
Industrial production of this compound emphasizes cost-effectiveness and reproducibility. Patent literature reveals adaptations of laboratory methods for large-scale synthesis, including continuous-flow reactors and catalyst recycling .
Key Industrial Modifications
-
Catalyst Systems : Heterogeneous catalysts (e.g., immobilized sulfonic acid resins) replace H2SO4 to simplify separation and reduce corrosion .
-
Reaction Temperature : Lower temperatures (40–60°C) are utilized in continuous systems to minimize side reactions, coupled with molecular sieves for in situ water removal .
-
Byproduct Management : Dithioester byproducts, formed via thiol group oxidation, are mitigated through inert atmospheres (e.g., nitrogen purge) .
Table 1: Comparison of Laboratory vs. Industrial Esterification Conditions
Parameter | Laboratory Scale | Industrial Scale |
---|---|---|
Catalyst | H2SO4 | Immobilized acid resins |
Temperature | 140–180°C (reflux) | 40–60°C (continuous) |
Reaction Time | 6–12 hours | 2–4 hours |
Yield | 60–75% | 80–85% |
Purification | Recrystallization | Vacuum distillation |
Challenges in Mercapto Group Stability
The thiol (-SH) group in this compound poses unique synthetic challenges due to its susceptibility to oxidation and nucleophilic side reactions. Studies on analogous compounds highlight strategies to preserve thiol integrity during synthesis :
Oxidation Mitigation
-
Inert Atmosphere : Conducting reactions under nitrogen or argon minimizes disulfide (R-S-S-R) formation .
-
Antioxidant Additives : Thiourea or ascorbic acid (0.1–0.5 wt%) suppress radical-mediated oxidation during purification .
Side Reaction Prevention
-
pH Control : Maintaining mildly acidic conditions (pH 4–5) reduces thiolate ion (RS−) formation, curtailing nucleophilic attacks on the ester group .
Alternative Synthetic Routes and Emerging Methods
While direct esterification dominates current practice, exploratory approaches have been investigated for niche applications:
Enzymatic Esterification
Lipase-catalyzed transesterification offers a solvent-free alternative, though yields remain suboptimal (30–40%) due to enzyme inhibition by the thiol group .
Thiolation of Preformed Esters
A two-step process involving:
-
Methyl picolinate synthesis via standard esterification.
-
Thiol group introduction via nucleophilic aromatic substitution with NaSH.
This method avoids direct handling of 3-mercaptopicolinic acid but requires stringent temperature control (<30°C) to prevent polysulfide formation .
Analytical Validation and Quality Control
Post-synthesis characterization ensures product integrity:
Properties
Molecular Formula |
C7H7NO2S |
---|---|
Molecular Weight |
169.20 g/mol |
IUPAC Name |
methyl 3-sulfanylpyridine-2-carboxylate |
InChI |
InChI=1S/C7H7NO2S/c1-10-7(9)6-5(11)3-2-4-8-6/h2-4,11H,1H3 |
InChI Key |
GYXRVHMNRSPGHY-UHFFFAOYSA-N |
Canonical SMILES |
COC(=O)C1=C(C=CC=N1)S |
Origin of Product |
United States |
Disclaimer and Information on In-Vitro Research Products
Please be aware that all articles and product information presented on BenchChem are intended solely for informational purposes. The products available for purchase on BenchChem are specifically designed for in-vitro studies, which are conducted outside of living organisms. In-vitro studies, derived from the Latin term "in glass," involve experiments performed in controlled laboratory settings using cells or tissues. It is important to note that these products are not categorized as medicines or drugs, and they have not received approval from the FDA for the prevention, treatment, or cure of any medical condition, ailment, or disease. We must emphasize that any form of bodily introduction of these products into humans or animals is strictly prohibited by law. It is essential to adhere to these guidelines to ensure compliance with legal and ethical standards in research and experimentation.