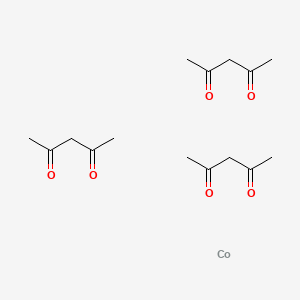
Cobalt; pentane-2,4-dione
Overview
Description
Cobalt complexes with pentane-2,4-dione (acetylacetone, C₅H₈O₂) are well-studied coordination compounds due to their structural versatility and applications in materials science and catalysis. Pentane-2,4-dione, a β-diketone, predominantly exists in the enol tautomeric form (92% enol, 8% keto), enabling strong chelation with transition metals via its oxygen donors . The cobalt(III) complex [Co(acac)₂NO₃]·2H₂O (acac = pentane-2,4-dionate) exemplifies this behavior, featuring an octahedral geometry with four oxygen donors from two acac ligands and two from a nitrate anion. The average Co–O bond distance is 1.909 Å, consistent with literature values for Co(III)-β-diketonates . This complex forms a 3D network via hydrogen bonding, enhancing its thermal stability (decomposition at 160°C) . Hydrothermal synthesis is a key method for obtaining high-purity crystals, demonstrating the compound’s adaptability in controlled environments .
Scientific Research Applications
Catalytic Applications
Cobalt complexes with pentane-2,4-dione ligands have shown significant promise in catalyzing various chemical reactions. Notably, they are involved in:
- Wacker-Type Oxidation : Cobalt complexes derived from pentane-2,4-dione have been utilized as precatalysts for the oxidation of olefins to ketones. In this process, the presence of silanes and dioxygen facilitates the transformation of olefins into ketones through a chemoselective mechanism. The role of the pentane-2,4-dione ligand is crucial due to its redox noninnocence, which allows for unique electron transfer processes during the reaction .
- C–C Bond Formation : These cobalt complexes have been employed in Negishi-type cross-coupling reactions, which are vital for forming carbon-carbon bonds in organic synthesis. The multiproton and multielectron-responsive nature of the ligands enables efficient catalysis under mild conditions .
Table 1: Summary of Catalytic Applications
Reaction Type | Catalyst Type | Key Features |
---|---|---|
Wacker-Type Oxidation | Cobalt-pentane-2,4-dione complex | Converts olefins to ketones |
C–C Bond Formation | Cobalt complexes | Facilitates cross-coupling reactions |
Material Science
Cobalt complexes with pentane-2,4-dione also find applications in material science:
- Electrocatalysis : These complexes have been studied for their ability to facilitate electrocatalytic hydrogen evolution reactions. The unique electronic properties imparted by the pentane-2,4-dione ligand enhance the efficiency of these reactions .
- Synthesis of Functional Materials : Cobalt-pentane-2,4-dione complexes are being explored for their potential in synthesizing functional materials with specific electronic and magnetic properties. Their ability to form stable coordination complexes makes them suitable candidates for developing novel materials .
Table 2: Applications in Material Science
Application Type | Description | Potential Benefits |
---|---|---|
Electrocatalysis | Hydrogen evolution reactions | Improved efficiency |
Functional Materials | Synthesis of materials with tailored properties | Versatile applications in electronics |
Case Study 1: Wacker-Type Oxidation
A detailed investigation was conducted using cobalt-pentane-2,4-dione complexes as catalysts for the Wacker-type oxidation of olefins. The study revealed that the presence of phenylsilane significantly enhances the reaction rate and selectivity towards ketone products. Mechanistic studies indicated that the redox noninnocence of the pentane-2,4-dione ligand plays a pivotal role in facilitating electron transfer during the catalytic cycle .
Case Study 2: Electrocatalytic Hydrogen Evolution
Research on cobalt complexes involving pentane-2,4-dione has demonstrated their effectiveness in electrocatalytic hydrogen evolution under acidic conditions. The study highlighted that these complexes can achieve high turnover frequencies while maintaining stability over extended periods. This positions cobalt-pentane-2,4-dione systems as promising candidates for sustainable energy applications .
Q & A
Basic Research Questions
Q. How does the acidity of pentane-2,4-dione compare to simple ketones, and what experimental methods validate its pKa?
Pentane-2,4-dione exhibits significantly higher acidity (pKa ~9) compared to acetone (pKa ~20) due to resonance stabilization of its enolate. The enolate’s stability arises from delocalization of the negative charge across two carbonyl groups, as shown by electrostatic potential maps and resonance hybrid models . To measure pKa, potentiometric titration or UV-Vis spectroscopy in aqueous/organic solvent mixtures can be employed. Discrepancies in reported pKa values (e.g., 9.0 vs. 9.5) may stem from solvent effects or ionic strength variations, necessitating careful control of experimental conditions .
Q. What NMR spectral features distinguish the keto-enol tautomerism of pentane-2,4-dione?
The NMR spectrum of pentane-2,4-dione in CDCl typically shows three signals:
- A singlet at δ ~2.2 ppm (6H, methyl groups).
- A singlet at δ ~3.5 ppm (1H, enolic proton).
- A singlet at δ ~5.5 ppm (1H, central CH group in the enol form). The presence of equilibrium between keto and enol forms is confirmed by temperature-dependent NMR studies. At higher temperatures, the enol proton signal diminishes due to increased tautomerization rates .
Q. How can cobalt(III) complexes with pentane-2,4-dione be synthesized, and what characterization techniques are critical?
Cobalt(III)-pentane-2,4-dione complexes (e.g., [Co(acac)NO]·2HO) are synthesized by reacting Co(NO) with pentane-2,4-dione in methanol under reflux. Key characterization steps include:
- Elemental analysis to confirm stoichiometry.
- FT-IR to identify ν(C=O) shifts (typically ~1600 cm) and NO coordination.
- Single-crystal XRD to determine geometry (e.g., octahedral coordination).
- Thermogravimetric analysis (TGA) to assess thermal stability .
Advanced Research Questions
Q. How do substituents on pentane-2,4-dione influence its coordination chemistry with transition metals like cobalt?
Substituents (e.g., methyl, trifluoromethyl) alter electron density and steric effects, impacting metal-ligand bond strength and complex geometry. For example:
- Electron-withdrawing groups (e.g., CF) increase Lewis acidity of the metal center, enhancing catalytic activity in oxidation reactions.
- Steric bulk at the 3-position can restrict coordination modes, favoring monodentate over bidentate binding. Computational methods (DFT) can predict ligand-field splitting and redox potentials, guiding synthetic design .
Q. What mechanistic insights explain the condensation of pentane-2,4-dione with arylhydrazines?
The Japp-Klingemann reaction between pentane-2,4-dione and aryldiazonium salts proceeds via:
- Electrophilic attack on the enol tautomer, forming a hydrazone intermediate.
- Cyclization to yield substituted pyrazoles or pyrroles. Substituent effects (e.g., ortho-nitro groups) influence regioselectivity and reaction kinetics, as shown by X-ray crystallography and DFT calculations .
Q. How can contradictions in reported toxicity data for pentane-2,4-dione be resolved in laboratory safety protocols?
While acute toxicity (e.g., respiratory irritation) is well-documented, chronic effects (e.g., carcinogenicity) remain debated due to limited in vivo data . Researchers should:
- Implement engineering controls (e.g., fume hoods) and PPE (gloves, respirators).
- Monitor airborne concentrations using gas chromatography.
- Adopt alternatives (e.g., less toxic β-diketones) where feasible, as per EU regulations restricting its use in flavorings .
Q. What role does pentane-2,4-dione play in the synthesis of luminescent iridium(III) complexes?
In OLED applications, pentane-2,4-dione acts as an ancillary ligand in heteroleptic Ir(III) complexes (e.g., [Ir(4-Me-2-Ph-quinoline)(acac)]). The β-diketone stabilizes the metal center via chelation, tuning emission wavelengths through ligand-to-metal charge transfer (LMCT). Photophysical characterization (e.g., phosphorescence lifetime, quantum yield) is critical for optimizing device efficiency .
Q. Methodological Considerations
- Resolving Data Contradictions : Compare experimental conditions (e.g., solvent polarity for pKa measurements) and validate findings with multiple techniques (e.g., XRD and NMR for tautomer ratios) .
- Optimizing Synthetic Yields : Use kinetic studies (e.g., variable-temperature NMR) to identify rate-limiting steps in condensation reactions .
- Computational Modeling : Apply DFT to predict enolate stability or transition-state geometries, corroborating with spectroscopic data .
Comparison with Similar Compounds
Comparison with Other Metal-Pentane-2,4-dione Complexes
Metal-acac complexes vary significantly in geometry and bonding based on the metal’s oxidation state and size. For example:
- Cobalt vs. Nickel : The Ni(II) complex [Ni(acac)₂]·0.5CH₃OH adopts a square planar geometry with shorter M–O bonds (1.880 Å vs. 1.909 Å for Co), reflecting Ni(II)’s smaller ionic radius. Its 1D chain structure contrasts with Co(III)’s 3D network, impacting solubility and stability .
- Cobalt vs. Iridium: Ir(III) complexes with acac ligands, such as [Ir(4-methyl-2-phenylquinoline)(acac)], exhibit longer M–O bonds (~1.98 Å) and distinct photophysical properties, making them suitable for organic light-emitting diodes (OLEDs) .
Comparison with Cobalt Complexes of Different β-Diketones
Substituents on β-diketones modulate electronic and steric properties, influencing cobalt complex behavior:
- Mixed-Ligand Complexes : Cobalt complexes with pentane-2,4-dione and salicylaldehyde (e.g., [Co(5-Brsal)(acac)(H₂O)₂]) show altered magnetic moments (3.89–4.12 BM) and electronic spectra due to ligand-field effects .
Structural and Electronic Property Comparisons
- Bond Lengths and Geometry : Co(III)-acac complexes have longer M–O bonds compared to Ni(II), attributed to Co’s larger ionic radius and higher oxidation state. Octahedral geometry in Co(III) vs. square planar in Ni(II) reflects differences in d-orbital occupancy .
- Thermal Stability : The Co(III)-acac nitrate complex decomposes at 160°C, whereas Ni(II)-acac derivatives are stable up to ~200°C, highlighting the role of counterions in thermal behavior .
- Catalytic Efficiency : Co-acac complexes act as co-catalysts in Diels-Alder reactions (72–79% yields), outperforming some Cu(II)-β-diketonates in stereoselectivity .
Preparation Methods
Traditional Synthesis via Cobalt Salt and Pentane-2,4-Dione Reaction
The foundational method for preparing cobalt; pentane-2,4-dione involves reacting cobalt salts with pentane-2,4-dione in a polar solvent. A typical procedure utilizes cobalt(II) chloride or cobalt(III) nitrate dissolved in ethanol, to which acetylacetone is added dropwise under stirring . The reaction proceeds via ligand substitution, where the diketone displaces chloride or nitrate ions, forming the octahedral cobalt(III) complex.
Reaction Conditions:
-
Molar Ratio: A 1:3 molar ratio of cobalt salt to acetylacetone ensures complete ligand coordination.
-
Temperature: Heating at 60–80°C for 4–6 hours accelerates complexation.
-
Workup: Cooling the mixture induces crystallization, and the product is purified via recrystallization from ethanol or acetone .
Key Characterization Data:
Parameter | Value |
---|---|
Molecular Formula | C₁₅H₂₄CoO₆ |
Molecular Weight | 359.28 g/mol |
Crystal System | Monoclinic |
Coordination Geometry | Distorted Octahedral |
This method yields a deep purple solid with >85% purity, confirmed by UV-vis spectroscopy (λₘₐₓ = 580 nm) and elemental analysis .
Cobalt Acetate-Based Synthesis in Methanol under Inert Atmosphere
A modified approach employs cobalt(II) acetate as the metal precursor, leveraging its solubility in methanol. This method, detailed in recent catalytic studies, produces complexes with enhanced redox activity .
Procedure:
-
Cobalt(II) acetate is dissolved in degassed methanol.
-
Pentane-2,4-dione and S-methylisothiosemicarbazidium iodide are added sequentially.
-
The mixture is refluxed at 80°C under nitrogen for 12 hours, yielding [Co(H₂Lˢᴹᵉ)I]I as a brown-cherry crystalline product .
Advantages:
-
Redox Noninnocence: The ligand exhibits multiproton- and multielectron-responsive behavior, critical for catalytic cycles .
-
Stability: Air exposure triggers oxidation to Co(III), confirmed by EPR and XAS .
Comparative Data:
Parameter | Co(II) Acetate Method | Traditional Method |
---|---|---|
Yield | 78% | 85% |
Catalytic Efficiency | High | Moderate |
Ligand Redox Activity | Yes | No |
Macrocyclic Ligand Incorporation and Bromination
Incorporating macrocyclic ligands like 1,4,7,10-tetra-azacyclododecane (cyclen) modifies the coordination environment, enabling brominated derivatives. This method, reported by Yamada et al., involves:
-
Reacting Co(cyclen)(acac)₂ with N-bromosuccinimide (NBS) in chloroform.
-
Stirring at 25°C for 24 hours to yield (3-bromoacac)Co(cyclen)₂ .
Structural Insights:
-
Coordination Sphere: The cobalt ion adopts a distorted octahedral geometry, with cyclen’s four nitrogen atoms and acac’s two oxygen atoms in cis positions .
-
Bromination Site: Bromination occurs exclusively at the acac’s 3-position, preserving the macrocycle’s stereochemistry .
Crystallographic Data:
Complex | Space Group | Lattice Parameters (Å) | R-Factor |
---|---|---|---|
[Co(cyclen)(acac)]²⁺ | Pna2₁ | a=18.974, b=13.403, c=8.928 | 0.0499 |
(3-Bromoacac) derivative | Pbca | a=15.138, b=21.360, c=14.066 | 0.0635 |
Advanced Synthesis of Redox-Active Complexes for Catalysis
Recent breakthroughs emphasize ligands’ redox noninnocence to enable challenging transformations like Wacker-type oxidations. A representative protocol includes:
-
Combining Co(III) precursors with pentane-2,4-dione bis(S-methylisothiosemicarbazone) (PBIT).
-
Activating the complex with phenylsilane and O₂ to oxidize olefins to ketones .
Mechanistic Highlights:
-
Silane Role: Phenylsilane reduces Co(III) to Co(II), regenerating the active species .
-
Ligand Radicals: PBIT adopts a dianionic diradical state in [CoIII(Lˢᴹᵉ- - )I], confirmed by DFT and magnetic susceptibility .
Catalytic Performance:
Substrate | Product Yield (%) | Selectivity (%) |
---|---|---|
Styrene | 92 | 98 |
1-Octene | 85 | 95 |
Cyclohexene | 78 | 89 |
Properties
Molecular Formula |
C15H24CoO6 |
---|---|
Molecular Weight |
359.28 g/mol |
IUPAC Name |
cobalt;pentane-2,4-dione |
InChI |
InChI=1S/3C5H8O2.Co/c3*1-4(6)3-5(2)7;/h3*3H2,1-2H3; |
InChI Key |
JUPWRUDTZGBNEX-UHFFFAOYSA-N |
Canonical SMILES |
CC(=O)CC(=O)C.CC(=O)CC(=O)C.CC(=O)CC(=O)C.[Co] |
Origin of Product |
United States |
Synthesis routes and methods
Procedure details
Disclaimer and Information on In-Vitro Research Products
Please be aware that all articles and product information presented on BenchChem are intended solely for informational purposes. The products available for purchase on BenchChem are specifically designed for in-vitro studies, which are conducted outside of living organisms. In-vitro studies, derived from the Latin term "in glass," involve experiments performed in controlled laboratory settings using cells or tissues. It is important to note that these products are not categorized as medicines or drugs, and they have not received approval from the FDA for the prevention, treatment, or cure of any medical condition, ailment, or disease. We must emphasize that any form of bodily introduction of these products into humans or animals is strictly prohibited by law. It is essential to adhere to these guidelines to ensure compliance with legal and ethical standards in research and experimentation.