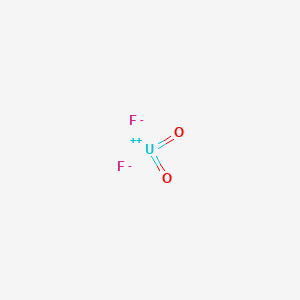
Uranyl fluoride
- Click on QUICK INQUIRY to receive a quote from our team of experts.
- With the quality product at a COMPETITIVE price, you can focus more on your research.
Overview
Description
Uranyl fluoride (UO₂F₂) is a significant compound in nuclear chemistry, primarily formed through the hydrolysis of uranium hexafluoride (UF₆) in the presence of moisture . This reaction releases hydrogen fluoride (HF), making UO₂F₂ a common byproduct in uranium enrichment facilities . Structurally, UO₂F₂ adopts a layered configuration where uranium is eight-coordinate, with two axial uranyl oxygen atoms and six equatorial fluoride ions bridging adjacent uranyl groups . This contrasts with most this compound complexes, which typically exhibit pentagonal-bipyramidal coordination (five equatorial ligands) .
UO₂F₂ is hygroscopic, readily adsorbing water to form crystal hydrates that influence its spectroscopic properties .
Preparation Methods
Synthetic Routes and Reaction Conditions
Uranyl fluoride can be synthesized through the reaction of uranium hexafluoride (UF6) with water or steam. The reaction is typically carried out under controlled conditions to ensure the formation of UO2F2:
UF6+2H2O→UO2F2+4HF
Industrial Production Methods
In industrial settings, the production of dioxouranium(2+);difluoride involves the controlled hydrolysis of uranium hexafluoride. This process is crucial in the nuclear fuel cycle, particularly in the conversion of uranium hexafluoride to uranium oxides, which are used as nuclear fuel.
Chemical Reactions Analysis
Hydration and Hydrolysis Reactions
Uranyl fluoride reacts with water vapor to form hydrolysis products, with humidity and temperature dictating the reaction pathway:
-
Primary Hydrolysis :
7UO2F2+11H2O→[(UO2F2)(H2O)]7⋅4H2O(hydrated uranyl fluoride)This metastable hydrate forms at moderate humidity (40% RH) and exhibits pentagonal coordination of the uranyl ion .
-
Secondary Hydrolysis :
[(UO2F2)(H2O)]7⋅4H2O→UO2(OH)2⋅nH2O(uranyl hydroxide)+HF
At ≥59% RH and 25–35°C, hydrated this compound degrades further:Prolonged exposure to 100% RH converts uranyl hydroxide into uranyl peroxide (UO₂O₂·4H₂O) .
Key Findings :
-
The reaction kinetics follow a denucleation mechanism mediated by water absorption .
-
Structural transitions involve a disordered intermediate phase ("L") with pentagonal uranyl coordination .
-
Fluorine loss is confirmed via EDS, with hydrated products showing no residual F .
Thermal Decomposition
At elevated temperatures, UO₂F₂ decomposes via:
3UO2F2→UF6+32U3O8+31O2(ΔH∘≈−5kcal/mol)
Temperature (°C) | Rate Constant (min⁻¹) | Activation Energy (kcal/mol) |
---|---|---|
700 | 7.6×10−5 | 50 |
800 | 7.8×10−4 |
Calcination in air (350–550°C) produces U₃O₈ as the dominant solid product, with residual UO₂F₂ detected via Raman spectroscopy until 525°C .
Reaction with Halogen Fluorides
UO₂F₂ reacts with chlorine trifluoride (ClF₃) at 50–150°C:
UO2F2+ClF3→UF6+ClO2F+ClF+O2+Cl2
Products :
Oxidation and Calcination Pathways
Calcination in air induces oxidation to uranium oxides:
3UO2F2+O2→U3O8+3F2
-
U₃O₈ formation begins at 350°C, with incomplete conversion even at 550°C.
-
Residual UO₂F₂ persists due to kinetic barriers, confirmed via Raman peaks at 845 cm⁻¹ (UO₂F₂) and 680 cm⁻¹ (U₃O₈) .
Morphological and Environmental Stability
-
Humidity Threshold : UO₂F₂ is stable at ≤32% RH but degrades at ≥59% RH .
-
Morphology Effects : Micro- and macrostructured UO₂F₂ (e.g., rods, plates) retain geometry during fluorination but exhibit surface roughening .
Data Tables
Table 2: Environmental Stability of UO₂F₂
Parameter | Stability Criterion | Observation |
---|---|---|
Relative Humidity (RH) | ≤32% RH | No degradation |
≥59% RH | Hydrolysis to UO₂(OH)₂·nH₂O | |
Temperature | 25–35°C | Degrades at high RH only |
Scientific Research Applications
Nuclear Science and Nonproliferation
Uranyl fluoride plays a significant role in nuclear science, particularly concerning nuclear nonproliferation efforts. Recent advancements have demonstrated the ability to simultaneously detect uranium isotopes and fluorine, which can provide critical insights into the origins and processes associated with nuclear materials. This capability is essential for monitoring nuclear activities and ensuring compliance with nonproliferation treaties .
Case Study: Detection Techniques
Researchers at Oak Ridge National Laboratory developed techniques that utilize laser-induced breakdown spectroscopy (LIBS) to map the distribution of fluorine in various samples, including biological materials like shark teeth. The findings suggest that this compound can indicate environmental conditions over time, thus extending its utility beyond nuclear applications into environmental science .
Environmental Monitoring
This compound's chemical properties allow it to be used in studies related to environmental contamination and the behavior of uranium in various ecosystems. Its stability under different environmental conditions makes it a useful marker for tracking uranium transport and transformation processes in soils and water systems.
Research Findings
A study highlighted the transformation of this compound when exposed to humid environments, leading to the formation of hydrated species that can alter its chemical behavior. This research is pivotal for understanding uranium's mobility in the environment and assessing the risks associated with uranium contamination .
Material Science
In material science, this compound is investigated for its potential applications in advanced materials development. Its unique structural properties make it a candidate for use in various high-tech applications, including:
- Nuclear Fuel Cycles : this compound serves as an intermediate in the nuclear fuel cycle, particularly during the conversion of uranium hexafluoride to other forms suitable for fuel fabrication.
- Hydrated Forms : Research into hydrated this compound has revealed insights into its stability and reactivity under different humidity levels, which can inform the design of safer storage methods for nuclear materials .
Toxicological Studies
Despite its applications, this compound poses significant health risks due to its nephrotoxic properties. Studies have shown that exposure can lead to kidney damage, necessitating careful handling and safety protocols in laboratories and industrial settings . Understanding these toxicological aspects is crucial for developing safety guidelines and protective measures.
Summary Table of Applications
Mechanism of Action
The mechanism of action of dioxouranium(2+);difluoride involves its interaction with various molecular targets. In nuclear chemistry, it acts as a precursor to other uranium compounds. Its interactions with ligands and reducing agents can lead to the formation of complexes and reduced uranium species, respectively. The pathways involved include hydrolysis, reduction, and complexation reactions.
Comparison with Similar Compounds
Comparison with Similar Uranyl Compounds
Structural and Coordination Chemistry
Uranyl Fluoride (UO₂F₂) vs. Uranyl Chloride (UO₂Cl₄²⁻) and Uranyl Nitrate (UO₂(NO₃)₂):
- Coordination Geometry :
- UO₂F₂ features an eight-coordinate uranium center (two O from uranyl, six F bridges) in a layered structure .
- Uranyl chloride ([UO₂Cl₄]²⁻) adopts a distorted octahedral geometry with four equatorial chloride ligands .
- Uranyl nitrate forms hexagonal-bipyramidal structures with six nitrate or water ligands in the equatorial plane .
- Bridging Behavior: In UO₂F₂, fluoride ions act as bridging ligands between uranyl units, forming extended layers .
Mixed-Ligand Complexes :
- Sulfato-fluoride complexes (e.g., K₂[UO₂F₂(SO₄)]·H₂O) exhibit structural diversity depending on the F:SO₄ ratio. At a 2:1 ratio, fluoride and sulfate share equatorial sites, forming polymeric chains .
- Carboxylato-fluoride complexes (e.g., Na₃[UO₂F₃(C₂O₄)]·6H₂O) combine fluoride bridges with organic ligands, creating 3D networks .
Table 1: Structural Comparison of Selected Uranyl Compounds
Spectroscopic and Thermodynamic Properties
Raman Spectroscopy :
- Fluoride’s lack of Raman activity makes it ideal for studying uranyl speciation without signal interference . In contrast, chloride and nitrate exhibit distinct Raman bands, complicating their analysis .
- UO₂F₂’s hydration state significantly affects its Raman spectrum, with crystal hydrates showing shifted uranyl symmetric stretch frequencies (~830 cm⁻¹) compared to anhydrous forms .
Fluorescence :
- Solid UO₂F₂ exhibits weak fluorescence (0.3% of uranyl nitrate’s intensity) due to quenching effects from crystal packing and hydration .
Thermodynamics :
- The temperature dependence of UO₂F₂ complexation in solution is extrapolated from uranyl chloride data, suggesting similar entropy-driven behavior .
Industrial Use :
- UO₂F₂ is critical in nuclear fuel processing, where UF₆ hydrolysis produces UO₂F₂, which is subsequently reduced to UO₂ for reactor fuel .
- Uranyl nitrate, by contrast, is used in analytical chemistry for ion detection (e.g., fluoride, chloride) .
Hazards :
- Both UF₆ and UO₂F₂ pose chemical risks due to HF release upon moisture exposure . Uranyl chloride and nitrate, while less volatile, present radiological hazards due to uranium’s radioactivity .
Q & A
Basic Research Questions
Q. What are the primary methods for synthesizing uranyl fluoride (UO₂F₂) in laboratory settings, and how do reaction conditions influence product purity?
this compound is commonly synthesized via the reaction of uranium hexafluoride (UF₆) with moisture , or through fluorination of uranium oxides (e.g., UO₃) using sulfur tetrafluoride (SF₄) or nitrogen trifluoride (NF₃). The latter method requires precise temperature control (e.g., 200–400°C) to avoid intermediate oxyfluoride formation . Product purity is highly sensitive to moisture levels and gas-phase reactant velocities; anhydrous conditions and controlled gas flow rates minimize hydrolysis byproducts .
Q. How can Raman spectroscopy be applied to characterize this compound complexes in aqueous solutions?
Raman spectroscopy, combined with quantum chemical calculations, is effective for identifying uranyl-fluoride speciation (e.g., UO₂F⁺, UO₂F₃⁻) by analyzing symmetric stretching (ν₁) modes of the UO₂²⁺ core. Shifts in ν₁ (~830–860 cm⁻¹) correlate with fluoride coordination numbers, while computational models (e.g., density functional theory) validate structural assignments .
Q. What are the key challenges in measuring fluorescence properties of solid-phase this compound compared to its solution-phase counterparts?
Solid-phase this compound exhibits minimal fluorescence (≤0.3% intensity of uranyl nitrate) due to quenching effects from crystal lattice rigidity and hydration states. In contrast, solution-phase fluorescence is enhanced by fluoride ion mobility, which stabilizes excited-state electronic transitions. Researchers must account for hydration history and crystallinity when interpreting solid-phase data .
Advanced Research Questions
Q. How can thermodynamic inconsistencies in this compound complexation constants be resolved across experimental and computational studies?
Discrepancies arise from varying ionic strengths, temperature regimes, and speciation models. A systematic approach involves:
- Conducting high-precision potentiometric titrations under inert atmospheres to minimize hydrolysis.
- Cross-validating results with spectroscopic techniques (e.g., EXAFS for structural data) .
- Applying activity coefficient corrections (e.g., Pitzer equations) for concentrated electrolytes .
Q. What experimental strategies address the lack of viscosity and thermal conductivity data for this compound solutions in reactor design applications?
For SHEBA II reactor modeling, researchers can:
- Use capillary viscometry with corrosion-resistant cells (e.g., Hastelloy C-276) for viscosity measurements.
- Employ transient hot-wire methods for thermal conductivity, ensuring calibration against reference fluids (e.g., molten salts) .
- Validate data against molecular dynamics simulations parameterized for UO₂²⁺-F⁻ interactions .
Q. Why do fluorescence spectra of this compound complexes show conflicting trends between ligand-field theory and experimental observations?
Theoretical models predict a ~2000 cm⁻¹ blueshift in electronic transitions due to equatorial fluoride ligands, but experimental spectra often show ligand-independent band positions. This contradiction arises from overlapping charge-transfer (CT) and f-f transitions. To resolve this:
- Use low-temperature luminescence spectroscopy to reduce spectral broadening.
- Apply parallel factor analysis (PARAFAC) to deconvolute overlapping bands .
- Compare with chloride/bromide analogues to isolate ligand-specific effects .
Q. How do kinetic parameters for this compound reactions (e.g., with SF₄) vary under non-ideal flow conditions, and how can these be modeled?
The reaction rate with SF₄ follows a 1.55-order dependence on gas partial pressure and is independent of flow velocity above a critical threshold (~5 cm/s). Activation energy (32 kcal/mol) suggests a rate-limiting step involving fluorine abstraction. Modeling requires:
- Fixed-bed reactor simulations with Arrhenius corrections for temperature gradients.
- In situ mass spectrometry to track intermediate species (e.g., UF₄) .
Q. Methodological Guidance
Q. What protocols ensure reproducibility in this compound synthesis and handling?
- Conduct reactions in rigorously dried systems (e.g., gloveboxes with <1 ppm H₂O).
- Characterize products via X-ray diffraction (XRD) and thermogravimetric analysis (TGA) to confirm phase purity .
- Store samples in sealed ampules under argon to prevent hygroscopic degradation .
Q. How can researchers mitigate self-absorption artifacts in this compound luminescence studies?
- Use thin-film samples or dilute solid dispersions (e.g., in KBr matrices).
- Apply front-face detection geometry to minimize path-length effects .
- Validate with time-resolved spectroscopy to differentiate radiative vs. non-radiative decay .
Q. What computational frameworks are recommended for modeling this compound charge-transfer dynamics?
Properties
Molecular Formula |
F2O2U |
---|---|
Molecular Weight |
308.025 g/mol |
IUPAC Name |
dioxouranium(2+);difluoride |
InChI |
InChI=1S/2FH.2O.U/h2*1H;;;/q;;;;+2/p-2 |
InChI Key |
KCKICANVXIVOLK-UHFFFAOYSA-L |
Canonical SMILES |
O=[U+2]=O.[F-].[F-] |
Origin of Product |
United States |
Disclaimer and Information on In-Vitro Research Products
Please be aware that all articles and product information presented on BenchChem are intended solely for informational purposes. The products available for purchase on BenchChem are specifically designed for in-vitro studies, which are conducted outside of living organisms. In-vitro studies, derived from the Latin term "in glass," involve experiments performed in controlled laboratory settings using cells or tissues. It is important to note that these products are not categorized as medicines or drugs, and they have not received approval from the FDA for the prevention, treatment, or cure of any medical condition, ailment, or disease. We must emphasize that any form of bodily introduction of these products into humans or animals is strictly prohibited by law. It is essential to adhere to these guidelines to ensure compliance with legal and ethical standards in research and experimentation.