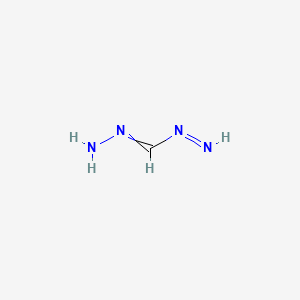
Diaminocarbodiimide
- Click on QUICK INQUIRY to receive a quote from our team of experts.
- With the quality product at a COMPETITIVE price, you can focus more on your research.
Overview
Description
Diaminocarbodiimides are a subclass of carbodiimides, characterized by two amine groups attached to the central carbodiimide moiety (N=C=N). These compounds are widely employed as coupling agents in organic synthesis, particularly in peptide and amide bond formation. Their mechanism involves activating carboxylic acids to form reactive intermediates (e.g., O-acylisourea), facilitating nucleophilic attack by amines . Common examples include dicyclohexylcarbodiimide (DCC), diisopropylcarbodiimide (DIC), and 1-ethyl-3-(3-dimethylaminopropyl)carbodiimide (EDCI). This article provides a detailed comparison of these compounds, emphasizing structural, functional, and application-based differences.
Scientific Research Applications
Chemical Properties and Mechanism
Diaminocarbodiimide is a carbodiimide compound characterized by the presence of two amine groups, which enhances its reactivity towards carboxylic acids. The primary mechanism of action involves the formation of an O-acylisourea intermediate, facilitating the coupling of amines with carboxylic acids to form amides. This reaction is particularly valuable in peptide synthesis and bioconjugation processes.
Biochemical Applications
Peptide Synthesis:
DACD is extensively used in the synthesis of peptides and proteins. It acts as a coupling agent, promoting the formation of peptide bonds between amino acids. This application is crucial in producing therapeutic peptides for drug development.
Crosslinking Agent:
In protein chemistry, DACD serves as a crosslinking agent, enabling the stabilization of protein structures. This property is utilized in creating biomaterials for drug delivery systems and tissue engineering applications. Studies have shown that DACD can effectively crosslink proteins, enhancing their stability and functionality in various assays .
Bioconjugation:
The ability of DACD to facilitate the conjugation of biomolecules has significant implications in developing diagnostic tools and therapeutics. For instance, it can be used to attach fluorescent labels to proteins or peptides, improving detection methods in research and clinical diagnostics .
Material Science Applications
Nanomaterials Functionalization:
DACD has been employed in the functionalization of nanomaterials, such as carbon nanotubes and graphene oxide. By attaching biological molecules to these nanomaterials via DACD-mediated reactions, researchers have enhanced their biocompatibility and targeted delivery capabilities .
Polymer Chemistry:
In polymer science, DACD is used to modify the surface properties of polymers. This modification can improve adhesion properties or introduce specific functionalities that are beneficial for applications in coatings and adhesives .
Pharmaceutical Applications
Drug Development:
The pharmaceutical industry leverages DACD for synthesizing complex drug molecules. Its ability to facilitate amide bond formation is particularly useful in creating prodrugs that enhance bioavailability or target specificity .
Anticancer Research:
Recent studies have indicated that DACD derivatives exhibit potential anticancer properties. Research has focused on synthesizing novel compounds using DACD that can inhibit cancer cell proliferation through various mechanisms, including apoptosis induction .
Case Study 1: Peptide Synthesis Using DACD
A study demonstrated the efficiency of DACD in synthesizing a therapeutic peptide for cancer treatment. The researchers reported a significant yield improvement compared to traditional coupling agents, highlighting DACD's effectiveness in forming stable peptide bonds under mild conditions .
Case Study 2: Functionalization of Graphene Oxide
In another study, researchers functionalized graphene oxide with biomolecules using DACD as a coupling agent. The functionalized graphene oxide exhibited enhanced cellular uptake and improved therapeutic efficacy in drug delivery systems targeting cancer cells .
Chemical Reactions Analysis
Reaction with Carboxylic Acids
EDC activates carboxyl groups to form reactive O-acylisourea intermediates, enabling amide bond formation with primary amines. This two-step mechanism proceeds as follows:
-
Carboxyl activation : The carbodiimide reacts with a carboxylic acid to form an O-acylisourea intermediate.
-
Nucleophilic substitution : A primary amine displaces the intermediate, forming an amide bond and releasing a water-soluble urea byproduct .
Key parameters :
-
Optimal pH: 4.5–7.2 (efficiency declines above pH 7.2 due to intermediate hydrolysis) .
-
Activation energy: 55.8±2.1kJ mol−1
for carbodiimide formation from isocyanates .
Kinetic Analysis of Amide Bond Formation
Studies using calorimetry and DFT calculations reveal:
-
Rate-determining step : Reaction between doubly protonated EDC (EDCIH22+
) and carboxylate anions . -
Second-order rate constant: kP=4.1×104M−1s−1
at 20°C in 1-methyl-2-pyrrolidinone (NMP) . -
Temperature dependence:
pH-Dependent Reactivity
EDC’s reactivity is highly pH-sensitive:
-
Low pH (4.5–5.5) : High EDCIH22+
concentration maximizes carboxyl activation . -
High pH (>7.2) : Reduced EDCIH22+
availability slows O-acylisourea formation, favoring hydrolysis .
Experimental validation :
-
Activation energy in water: 51.9±6.1kJ mol−1
(matches DFT predictions) . -
Rate constant in organic solvents: 105×
higher than in aqueous media .
Side Reactions and Mitigation
Competing pathways include:
-
O-acylisourea hydrolysis : Regenerates carboxylic acid, reducing yield .
-
Intramolecular cyclization : Observed in amino acids with internal amines (e.g., forming diamides) .
Solutions :
Q & A
Basic Research Questions
Q. What are the standard synthetic routes for diaminocarbodiimide, and how can purity be validated experimentally?
Diaminocarbodiimides are typically synthesized via carbodiimidization reactions between amines and carbodiimide precursors. For example, DeTar and Silverstein (1966) demonstrated that reactions of dicyclohexylcarbodiimide with carboxylic acids in the presence of amines yield stable intermediates . To validate purity, researchers should employ tandem techniques:
- NMR spectroscopy to confirm structural integrity (e.g., characteristic carbodiimide peaks at ~2100–2150 cm⁻¹ in IR).
- HPLC-MS to detect byproducts like ureas or thioureas.
- Elemental analysis to verify stoichiometric ratios of C, H, and N .
Q. How can researchers optimize reaction conditions for this compound-mediated couplings in peptide synthesis?
Key variables include solvent polarity, temperature, and stoichiometry. A methodological approach involves:
- Screening solvents (e.g., DMF vs. THF) to balance reactivity and side reactions.
- Using a central composite design (CCD) to model interactions between temperature (20–60°C) and molar ratios (1:1 to 1:3).
- Monitoring reaction progress via thin-layer chromatography (TLC) or in situ FTIR to detect carbodiimide consumption .
Q. What spectroscopic techniques are most reliable for characterizing this compound derivatives?
- ¹H/¹³C NMR : Identify amine proton environments and carbodiimide carbons (δ ~140–160 ppm).
- X-ray crystallography : Resolve steric effects in bulky derivatives (e.g., cyclohexyl vs. aryl substituents).
- Raman spectroscopy : Detect vibrational modes obscured in IR by solvent interference .
Advanced Research Questions
Q. How can computational methods resolve contradictions in experimental data on this compound reactivity?
Discrepancies in reaction outcomes (e.g., unexpected regioselectivity) may arise from solvation effects or transition-state stabilization. Researchers should:
- Perform density functional theory (DFT) calculations (e.g., B3LYP/6-31G*) to model transition states and compare with experimental kinetics.
- Use molecular dynamics (MD) simulations to assess solvent interactions (e.g., aqueous vs. aprotic media).
- Cross-validate with Arrhenius plots to reconcile computational activation energies with empirical data .
Q. What strategies mitigate this compound hydrolysis during long-term storage?
Hydrolysis to ureas is a critical stability challenge. Methodological solutions include:
- Lyophilization under inert gas (argon) to remove residual moisture.
- Formulating with moisture scavengers (e.g., molecular sieves or anhydrous MgSO₄).
- Conducting accelerated stability studies (40°C/75% RH) with periodic HPLC analysis to quantify degradation .
Q. How should researchers design experiments to probe this compound’s role in supramolecular assembly?
- Isothermal titration calorimetry (ITC) : Quantify binding affinities with host molecules (e.g., cyclodextrins).
- Small-angle X-ray scattering (SAXS) : Monitor self-assembly kinetics in real time.
- Variable-temperature NMR : Detect dynamic conformational changes in solution .
Q. Methodological Frameworks for Data Analysis
Q. What statistical approaches are suitable for analyzing contradictory catalytic efficiency data in this compound systems?
- Apply multivariate regression to isolate variables (e.g., solvent polarity, substituent electronics).
- Use Bland-Altman plots to assess agreement between replicate experiments.
- Employ meta-analysis (PRISMA guidelines) to synthesize literature data and identify trends .
Q. How can mixed-methods research designs enhance mechanistic studies of this compound reactions?
Combine quantitative kinetics (e.g., stopped-flow spectroscopy) with qualitative mechanistic probes (e.g., isotopic labeling or trapping experiments). This triangulation addresses limitations of single-method approaches .
Q. Guidance for Research Design
Q. What criteria (e.g., FINER) should guide hypothesis formulation for this compound-based studies?
Ensure hypotheses are:
- Feasible : Access to specialized equipment (e.g., gloveboxes for moisture-sensitive reactions).
- Novel : Addressing gaps, such as understudied substituent effects on reactivity.
- Ethical : Proper disposal protocols for toxic byproducts (e.g., cyanamide derivatives) .
Q. How can researchers align this compound studies with reproducibility standards?
Comparison with Similar Compounds
Structural and Chemical Properties
- Dicyclohexylcarbodiimide (DCC) : Features two cyclohexyl groups. Its hydrophobic nature limits solubility in polar solvents, requiring use in dichloromethane or dimethylformamide (DMF). The byproduct, dicyclohexylurea, is insoluble and complicates purification .
- Diisopropylcarbodiimide (DIC) : Contains isopropyl groups, offering better solubility in organic solvents compared to DCC. Its liquid state simplifies handling, and the urea byproduct is more soluble, enabling efficient solid-phase synthesis .
- EDCI: Modified with an ethyl-dimethylaminopropyl group, enhancing water solubility. Often used with hydroxybenzotriazole (HOBt) to stabilize intermediates and improve coupling efficiency in solution-phase reactions .
Reactivity and Byproduct Management
- DCC : High reactivity but forms insoluble dicyclohexylurea, necessitating filtration or extraction. This limits its utility in large-scale synthesis .
- DIC : Generates soluble urea derivatives, making it preferable for automated solid-phase peptide synthesis (SPPS). Lower toxicity compared to DCC .
- EDCI : Paired with HOBt, it forms water-soluble urea, streamlining purification. Ideal for solution-phase synthesis of sensitive compounds .
Table 1: Comparative Overview of Diaminocarbodiimides
Property | DCC | DIC | EDCI |
---|---|---|---|
Molecular Weight | 206.33 g/mol | 126.20 g/mol | 191.70 g/mol |
Solubility | Low in polar solvents | Moderate in organic solvents | High in polar solvents |
Byproduct Solubility | Insoluble | Soluble | Soluble (with HOBt) |
Common Applications | Peptide synthesis, analytics | Solid-phase synthesis | Bioconjugation, hybrid molecules |
Toxicity | Skin irritant | Lower toxicity | Moderate |
Research Findings
- DCC vs. DIC in SPPS : A study by Drabarek & Holodowicz (1983) demonstrated that DIC achieved higher yields (89–93%) in SPPS compared to DCC (78–85%), attributed to reduced steric hindrance and better byproduct solubility .
- EDCI in Hybrid Synthesis: EDCI enabled efficient coupling of 5-aminoindole with carboxylic acids, producing indole–caffeic acid hybrids with radical scavenging activity (IC₅₀: 12–45 μM) .
- Analytical Use of DCC: Ozawa & Tsukioka (1987) utilized DCC for derivatizing sodium monofluoroacetate in water, achieving detection limits of 0.1 ppm via gas chromatography .
Preparation Methods
Carbodiimide-Mediated Peptide Synthesis
Reaction Mechanism and Reagent Selection
The carbodiimide method involves activating carboxyl groups for amide bond formation. Dicyclohexylcarbodiimide (DCC) or 1-ethyl-3-(3-dimethylaminopropyl)carbodiimide (EDC) serves as the primary coupling agent, with 1-hydroxy-benzotriazole (HOBt) as an additive to suppress racemization and improve yields . The mechanism proceeds via an O-acylisourea intermediate, which reacts with amines to form peptides.
Key Reaction Steps:
-
Activation : Carbodiimide reacts with the carboxyl group to form an active ester.
-
Nucleophilic Attack : The amine attacks the activated carbonyl, releasing a urea byproduct.
-
Additive Role : HOBt stabilizes the intermediate, preventing side reactions like dehydration of asparagine or glutamine residues .
Optimization Strategies
-
Temperature Control : Reactions performed at 0–30°C minimize epimerization .
-
Solvent Selection : Dichloromethane (DCM) or dimethylformamide (DMF) enhances solubility of protected amino acids.
-
Stoichiometry : A 1:1:1 molar ratio of carboxyl component, amine, and carbodiimide ensures complete conversion .
Case Study: Synthesis of Z-Asn-Leu-OCl
Using DCC/HOBt, Z-protected asparagine reacted with leucine methyl ester at 25°C for 24 hours, yielding 85% pure product . Without HOBt, yields dropped to 39–45% due to nitrile byproduct formation.
Industrial-Scale Synthesis of EDC Hydrochloride
Multi-Step Synthesis Protocol
The Chinese patent CN109369458A outlines a seven-step process for 1-ethyl-3-(3-dimethylaminopropyl)carbodiimide hydrochloride (EDC·HCl) :
Stepwise Procedure:
-
EDTC Formation : Ethylamine and carbon disulfide react in alkaline conditions (10–30°C, 0.5–1 h).
-
EITC Synthesis : Trichloromethyl chloroformate (TCF) is added to EDTC at 15–30°C.
-
Distillation : Crude EITC is purified via oil bath distillation (100–150°C).
-
Intermediate Formation : EITC reacts with N,N-dimethyl-1,3-propanediamine (0–30°C).
-
Oxidation : TCF oxidizes the intermediate to EDC.
-
Extraction : Hexane or petroleum ether isolates EDC.
-
Salt Formation : EDC undergoes HCl exchange in aprotic solvents (10–30°C).
Performance Metrics
Step | Reactants | Conditions | Yield (%) | Purity (%) |
---|---|---|---|---|
1 | Ethylamine, CS₂ | 25°C, 1 h | 89 | >99 |
2 | EDTC, TCF | 20°C, 2.5 h | 90 | >99 |
7 | EDC, HCl | 20°C, 1 h | 89 | 99.5 |
Key Advantages :
-
High yields (>89%) at each stage.
-
Scalable to industrial production with minimal organic solvent use .
Comparative Analysis of Methods
Efficiency and Limitations
Parameter | Carbodiimide/HOBt | EDC·HCl Synthesis |
---|---|---|
Yield | 73–85% | 89–90% |
Purity | >95% | >99% |
Scalability | Lab-scale | Industrial |
Byproducts | Nitriles, imides | Minimal |
Emerging Innovations
Properties
Molecular Formula |
CH4N4 |
---|---|
Molecular Weight |
72.07 g/mol |
IUPAC Name |
formazan |
InChI |
InChI=1S/CH4N4/c2-4-1-5-3/h1-2H,3H2 |
InChI Key |
VMGAPWLDMVPYIA-UHFFFAOYSA-N |
Canonical SMILES |
C(=NN)N=N |
Origin of Product |
United States |
Disclaimer and Information on In-Vitro Research Products
Please be aware that all articles and product information presented on BenchChem are intended solely for informational purposes. The products available for purchase on BenchChem are specifically designed for in-vitro studies, which are conducted outside of living organisms. In-vitro studies, derived from the Latin term "in glass," involve experiments performed in controlled laboratory settings using cells or tissues. It is important to note that these products are not categorized as medicines or drugs, and they have not received approval from the FDA for the prevention, treatment, or cure of any medical condition, ailment, or disease. We must emphasize that any form of bodily introduction of these products into humans or animals is strictly prohibited by law. It is essential to adhere to these guidelines to ensure compliance with legal and ethical standards in research and experimentation.