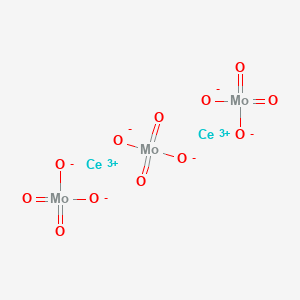
Cerous molybdate
Overview
Description
Cerous molybdate (Ce₂(MoO₄)₃) is a rare-earth molybdate compound characterized by its unique structural and chemical properties. Molybdates, in general, are oxyanionic compounds where molybdenum (Mo) is in the +6 oxidation state, coordinated with oxygen in tetrahedral (MoO₄²⁻) or polymeric configurations.
Preparation Methods
Synthetic Routes and Reaction Conditions: Cerium molybdenum oxide can be synthesized through several methods. One common approach involves the co-precipitation of cerium and molybdenum salts. The process typically includes the following steps:
- Dissolving cerium nitrate and ammonium molybdate in water.
- Adjusting the pH of the solution to induce precipitation.
- Filtering, washing, and drying the precipitate to obtain cerium molybdenum oxide .
Industrial Production Methods: In industrial settings, cerium molybdenum oxide is often produced using high-temperature solid-state reactions. This method involves mixing cerium oxide and molybdenum oxide powders, followed by calcination at elevated temperatures to form the desired compound .
Chemical Reactions Analysis
Types of Reactions: Cerium molybdenum oxide undergoes various chemical reactions, including:
Oxidation: It can act as an oxidizing agent in redox reactions.
Reduction: Under certain conditions, it can be reduced to lower oxidation states.
Substitution: It can participate in substitution reactions where one of its components is replaced by another element or compound.
Common Reagents and Conditions:
Oxidation: Common oxidizing agents include hydrogen peroxide and potassium permanganate.
Reduction: Reducing agents such as hydrogen gas or hydrazine can be used.
Substitution: Reactions often occur in the presence of acids or bases to facilitate the exchange of ions.
Major Products: The products of these reactions depend on the specific conditions and reagents used. For example, oxidation reactions may yield higher oxidation state compounds, while reduction reactions can produce lower oxidation state species .
Scientific Research Applications
Photocatalytic Applications
Photocatalysis is one of the most prominent applications of cerous molybdate. The compound has shown remarkable efficiency in the degradation of organic pollutants and dyes under UV light.
- Efficiency in Dye Degradation : Research indicates that this compound nanoparticles can effectively degrade dyes such as Fuschin and crystal violet. For instance, a study reported a degradation efficiency of 92% for Fuschin dye within 90 minutes under solar light irradiation . Another study demonstrated an 89% degradation rate for crystal violet after five hours of visible light exposure .
- Antimony Doping : The incorporation of antimony into this compound has been shown to enhance its photocatalytic properties. An Sb-doped variant achieved a photocatalytic efficiency of 85.8% against diclofenac potassium within 180 minutes of UV exposure, with the reaction following pseudo-first-order kinetics .
Corrosion Inhibition
This compound serves as an effective corrosion inhibitor for metallic alloys, particularly aluminum alloys.
- Performance in Coatings : Studies have demonstrated that this compound can improve the corrosion resistance of epoxy-coated aluminum alloys. The addition of this compound containers in coatings exposed to saline environments significantly enhanced their performance over 28 days .
- Mechanism : The protective mechanism is attributed to the formation of a stable oxide layer on the metal surface, which reduces the rate of corrosion in aggressive environments.
Dielectric Properties
This compound exhibits interesting dielectric properties , making it suitable for applications in electronic devices.
- Dielectric Constant : Research has shown that doping this compound with antimony leads to an increase in its dielectric constant and a decrease in loss tangent, indicating improved energy storage capabilities . For example, the dielectric constant was recorded at at 20 Hz for doped samples.
- Charge Storage Applications : The enhanced dielectric properties suggest potential applications in charge storage devices, where efficient energy retention is critical.
Data Summary
Mechanism of Action
The mechanism by which cerium molybdenum oxide exerts its effects involves its redox activity. The compound can switch between different oxidation states, primarily Ce3+ and Ce4+, allowing it to participate in redox reactions. This redox cycling enables it to neutralize reactive oxygen species, making it effective as an antioxidant . Additionally, its ability to interact with bacterial cell walls contributes to its antibacterial properties .
Comparison with Similar Compounds
Comparison with Similar Molybdate Compounds
Structural and Thermal Stability
- Yttrium Molybdate (Y₂Mo₃O₁₂): Under high pressure (up to 4.9 GPa), yttrium molybdate transitions from an orthorhombic Pbcn phase to a monoclinic P21/a phase, which remains stable without further abrupt transitions. In contrast, chromium molybdate (Cr₂Mo₃O₁₂) shows subtle structural distortions under compression, similar to Fe₂Mo₃O₁₂ and Al₂Mo₃O₁² . Cerous molybdate’s high-pressure behavior is less documented, but its rare-earth composition may confer distinct compressibility trends compared to transition-metal molybdates.
- Uranyl Molybdates : Uranyl molybdates (e.g., Cu(UO₂)(MoO₄)₂·6H₂O) exhibit diverse topologies due to uranyl (UO₂²⁺) and molybdate linkages. These structures often feature layered or framework architectures, differing from this compound’s simpler rare-earth coordination .
Table 1: Structural Stability Under High Pressure
Compound | Phase Transition Pressure | Stability Characteristics |
---|---|---|
Y₂Mo₃O₁₂ | 0.13 GPa | Stable monoclinic P21/a phase |
Cr₂Mo₃O₁₂ | 8.9 GPa | Subtle distortion, no abrupt change |
Ce₂(MoO₄)₃ (predicted) | N/A | Likely high stability due to Ce³⁺ |
Corrosion Inhibition
- Sodium Molybdate vs. Sodium Tungstate: Both inhibit corrosion in alkaline environments, with tungstate (WO₄²⁻) slightly outperforming molybdate (MoO₄²⁻) in reducing corrosion current density (Icorr: 1.2 µA/cm² vs. 1.5 µA/cm²) . This compound’s inhibition mechanism may differ due to Ce³⁺’s ability to form protective oxide films, combining anodic and cathodic inhibition .
- Comparison with Chromate and Vanadate : Chromate (CrO₄²⁻) and vanadate (VO₄³⁻) are potent inhibitors but face environmental restrictions. Molybdates, including this compound, offer a less toxic alternative with comparable efficiency in specific pH ranges .
Table 2: Corrosion Inhibition Efficiency
Inhibitor | Icorr (µA/cm²) | Mechanism | Environmental Impact |
---|---|---|---|
Sodium Molybdate | 1.5 | Mixed oxide film formation | Low toxicity |
Sodium Tungstate | 1.2 | Competitive adsorption | Moderate toxicity |
This compound | ~1.4 (predicted) | Ce³⁺ oxide film + MoO₄²⁻ | Low toxicity |
Catalytic and Polymerization Activity
- Sodium Molybdate in Catalysis : Sodium molybdate catalyzes catechol polymerization at pH 2, outperforming uranyl nitrate (pH 6 required) due to strong aromatic interactions . This compound’s catalytic activity may be enhanced by Ce³⁺’s redox activity, enabling applications in oxidative reactions.
Biological Activity
Cerous molybdate, specifically cerium molybdate (Ce(MoO)), has garnered attention in various fields due to its unique biological activities and potential applications in environmental remediation, catalysis, and nanotechnology. This article explores the biological activity of this compound, including its antibacterial, antiviral properties, and photocatalytic efficiency, supported by case studies and research findings.
Overview of this compound
This compound is a metal molybdate compound characterized by its semiconductive properties. It can exist in different crystalline forms, including monoclinic and tetragonal structures, depending on the synthesis conditions such as temperature and precursor materials used. The biological activity of this compound can be influenced by its structural properties, particle size, and the presence of dopants.
Antibacterial and Antiviral Activity
Recent studies have demonstrated that this compound exhibits significant antibacterial and antiviral properties. For instance:
- Antibacterial Activity : Research has shown that cerium molybdate nanoparticles effectively inhibit the growth of Escherichia coli and Staphylococcus aureus. The mechanism involves both direct contact with bacterial cells and the release of dissolved ions that exert antimicrobial effects .
- Antiviral Activity : this compound has also been evaluated for its antiviral properties against bacteriophages such as Qβ and Φ6. The antiviral efficacy is attributed to the inactivation of alkaline phosphatase enzyme proteins on these materials, suggesting a dual mechanism involving both surface interaction and ion release .
Photocatalytic Properties
The photocatalytic activity of this compound has been extensively studied, particularly in the degradation of organic pollutants under UV light. Key findings include:
- Efficiency : Pristine cerium molybdate demonstrated a photocatalytic efficiency of approximately 85.8% for the degradation of diclofenac potassium within 180 minutes of UV exposure. This efficiency was significantly enhanced through doping with antimony (Sb), which improved charge separation and reduced recombination rates .
- Kinetics : The photocatalytic reaction followed pseudo-first-order kinetics with an apparent rate constant of 0.0105 min. The introduction of Sb into the cerium molybdate lattice not only improved photocatalytic performance but also enhanced dielectric properties, making it suitable for applications in charge storage devices .
Synthesis Methods
This compound can be synthesized using various methods that affect its morphology and biological activity:
- Co-precipitation Method : This method allows for controlled doping and results in smaller particle sizes conducive to enhanced biological activity .
- Solvothermal Method : This approach yields nanostructures with improved surface area and reactivity, further enhancing photocatalytic efficiency .
Case Study 1: Antibacterial Efficacy
A study investigated the antibacterial effects of cerium molybdate nanoparticles against E. coli. The results indicated a significant reduction in bacterial viability upon exposure to these nanoparticles, confirming their potential as antibacterial agents in medical applications.
Case Study 2: Photocatalytic Degradation
In another study focusing on wastewater treatment, cerium molybdate showed remarkable efficiency in degrading pharmaceutical contaminants like diclofenac. The study highlighted the role of UV light in activating the photocatalyst, leading to effective pollutant breakdown.
Data Summary
Property | Value/Description |
---|---|
Crystalline Structure | Monoclinic / Tetragonal |
Antibacterial Activity | Effective against E. coli and S. aureus |
Antiviral Activity | Inactivation of bacteriophages Qβ and Φ6 |
Photocatalytic Efficiency | 85.8% degradation of diclofenac potassium |
Kinetics | Pseudo-first-order with k = 0.0105 min |
Q & A
Basic Research Questions
Q. What experimental parameters are critical for optimizing the synthesis of cerous molybdate (Ce₂(MoO₄)₃) to achieve phase purity?
- Methodological Answer :
- Control pH and temperature : Adjusting pH (e.g., acidic conditions for molybdate ion stability) and reaction temperature (e.g., hydrothermal synthesis at 120–200°C) directly influences crystallinity and phase formation. Use X-ray diffraction (XRD) to validate phase purity .
- Precursor stoichiometry : Precise molar ratios of cerium (Ce³⁺) to molybdate (MoO₄²⁻) prevent secondary phases. Thermogravimetric analysis (TGA) can monitor decomposition intermediates .
- Post-synthesis treatments : Annealing in controlled atmospheres (e.g., air or inert gas) modifies defect structures. Pair with Raman spectroscopy to detect oxygen vacancy-related bands .
Q. Which characterization techniques are most effective for analyzing the structural and compositional properties of this compound?
- Methodological Answer :
- XRD and Rietveld refinement : Quantify lattice parameters and identify impurity phases .
- FTIR and Raman spectroscopy : Probe Mo-O and Ce-O bonding environments. For example, Mo–O–Mo symmetric stretching in molybdate anions appears at 800–900 cm⁻¹ in Raman spectra .
- SEM-EDS : Map elemental distribution and confirm stoichiometry. Cross-validate with inductively coupled plasma optical emission spectroscopy (ICP-OES) for quantitative analysis .
Q. How can researchers design experiments to assess the thermal stability of this compound under varying environmental conditions?
- Methodological Answer :
- Controlled thermal cycling : Use TGA-DSC to monitor weight loss and phase transitions up to 1000°C. Compare results with in-situ XRD to correlate structural changes with thermal events .
- Humidity exposure tests : Measure hygroscopicity by exposing samples to 30–90% relative humidity and track mass changes. Pair with FTIR to detect hydroxyl group formation .
Advanced Research Questions
Q. How can spectral data contradictions (e.g., NMR or XRD) in this compound studies be resolved?
- Methodological Answer :
- Multi-technique validation : For NMR, compare chemical shifts (e.g., ¹H and ¹³C) with reference complexes (e.g., galactitol-molybdate systems) to confirm coordination geometry .
- Ab-initio modeling : Use density functional theory (DFT) to simulate XRD patterns or NMR spectra and match experimental data. Address discrepancies arising from polymorphism or hydration states .
- Error analysis : Quantify instrumental precision (e.g., XRD angle resolution) and sample heterogeneity (e.g., via SEM particle size distribution) .
Q. What mechanistic insights can be derived from studying this compound’s role in catalytic or photocatalytic reactions?
- Methodological Answer :
- Kinetic isotope effects (KIE) : Replace H₂O with D₂O in hydrolysis reactions to identify rate-determining steps involving proton transfer .
- In-situ XAFS : Track changes in Ce and Mo oxidation states during catalysis. For example, Ce³⁺ ↔ Ce⁴⁺ redox pairs can be monitored at the L₃-edge .
- Surface-sensitive techniques : Employ XPS or Auger electron spectroscopy (AES) to correlate surface defects (e.g., oxygen vacancies) with catalytic activity .
Q. How can computational models improve the understanding of this compound’s electronic structure and defect chemistry?
- Methodological Answer :
- DFT bandgap calculations : Compare computed bandgaps with UV-Vis diffuse reflectance spectra. Adjust Hubbard U parameters for Ce 4f electrons to improve accuracy .
- Defect formation energies : Simulate Schottky or Frenkel defects using VASP or Quantum ESPRESSO. Validate with positron annihilation spectroscopy .
Q. What experimental designs are suitable for investigating ion-exchange or intercalation properties of this compound?
- Methodological Answer :
- Electrochemical titration : Use a three-electrode cell with this compound as the working electrode. Monitor ion uptake/release via cyclic voltammetry (CV) in Na₂SO₄ or KCl electrolytes .
- In-situ XRD/PXRD : Capture structural expansion/contraction during ion intercalation. Pair with ICP-MS to quantify ion diffusion coefficients .
Q. Addressing Data Contradictions and Reproducibility
Q. Why do reported bandgap values for this compound vary across studies, and how can this be mitigated?
- Methodological Answer :
- Standardize measurement protocols : Use identical light sources and monochromators for UV-Vis. Calibrate with reference materials (e.g., TiO₂) .
- Sample preparation : Ensure consistent particle size via ball milling or sieving. Agglomeration can scatter light and inflate bandgap estimates .
Q. How can researchers ensure reproducibility in synthesizing this compound with consistent morphology?
- Methodological Answer :
- Document reaction kinetics : Record time-resolved pH and temperature data. Use statistical software (e.g., SAS or R) to model nucleation rates .
- Adopt FAIR principles : Publish raw XRD, SEM, and spectroscopic data in repositories like Zenodo with detailed metadata .
Properties
IUPAC Name |
cerium(3+);dioxido(dioxo)molybdenum | |
---|---|---|
Details | Computed by Lexichem TK 2.7.0 (PubChem release 2021.05.07) | |
Source | PubChem | |
URL | https://pubchem.ncbi.nlm.nih.gov | |
Description | Data deposited in or computed by PubChem | |
InChI |
InChI=1S/2Ce.3Mo.12O/q2*+3;;;;;;;;;;6*-1 | |
Details | Computed by InChI 1.0.6 (PubChem release 2021.05.07) | |
Source | PubChem | |
URL | https://pubchem.ncbi.nlm.nih.gov | |
Description | Data deposited in or computed by PubChem | |
InChI Key |
OEVVRXVLVQWRBF-UHFFFAOYSA-N | |
Details | Computed by InChI 1.0.6 (PubChem release 2021.05.07) | |
Source | PubChem | |
URL | https://pubchem.ncbi.nlm.nih.gov | |
Description | Data deposited in or computed by PubChem | |
Canonical SMILES |
[O-][Mo](=O)(=O)[O-].[O-][Mo](=O)(=O)[O-].[O-][Mo](=O)(=O)[O-].[Ce+3].[Ce+3] | |
Details | Computed by OEChem 2.3.0 (PubChem release 2021.05.07) | |
Source | PubChem | |
URL | https://pubchem.ncbi.nlm.nih.gov | |
Description | Data deposited in or computed by PubChem | |
Molecular Formula |
Ce2Mo3O12 | |
Details | Computed by PubChem 2.1 (PubChem release 2021.05.07) | |
Source | PubChem | |
URL | https://pubchem.ncbi.nlm.nih.gov | |
Description | Data deposited in or computed by PubChem | |
Molecular Weight |
760.1 g/mol | |
Details | Computed by PubChem 2.1 (PubChem release 2021.05.07) | |
Source | PubChem | |
URL | https://pubchem.ncbi.nlm.nih.gov | |
Description | Data deposited in or computed by PubChem | |
Physical Description |
Pellets or Large Crystals | |
Record name | Cerium molybdenum oxide (Ce2Mo3O12) | |
Source | EPA Chemicals under the TSCA | |
URL | https://www.epa.gov/chemicals-under-tsca | |
Description | EPA Chemicals under the Toxic Substances Control Act (TSCA) collection contains information on chemicals and their regulations under TSCA, including non-confidential content from the TSCA Chemical Substance Inventory and Chemical Data Reporting. | |
CAS No. |
13454-70-1 | |
Record name | Cerium molybdenum oxide (Ce2Mo3O12) | |
Source | EPA Chemicals under the TSCA | |
URL | https://www.epa.gov/chemicals-under-tsca | |
Description | EPA Chemicals under the Toxic Substances Control Act (TSCA) collection contains information on chemicals and their regulations under TSCA, including non-confidential content from the TSCA Chemical Substance Inventory and Chemical Data Reporting. | |
Disclaimer and Information on In-Vitro Research Products
Please be aware that all articles and product information presented on BenchChem are intended solely for informational purposes. The products available for purchase on BenchChem are specifically designed for in-vitro studies, which are conducted outside of living organisms. In-vitro studies, derived from the Latin term "in glass," involve experiments performed in controlled laboratory settings using cells or tissues. It is important to note that these products are not categorized as medicines or drugs, and they have not received approval from the FDA for the prevention, treatment, or cure of any medical condition, ailment, or disease. We must emphasize that any form of bodily introduction of these products into humans or animals is strictly prohibited by law. It is essential to adhere to these guidelines to ensure compliance with legal and ethical standards in research and experimentation.