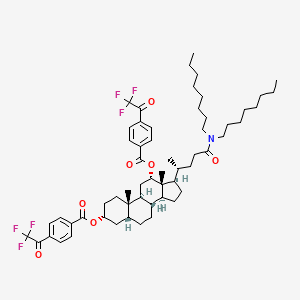
Carbonate ionophore VII
Overview
Description
Carbonate Ionophore VII, commercially available as a critical component in ion-selective electrodes (ISEs), is a tweezer-type ionophore derived from deoxy-3,12-bis(trifluoroacetyl) cholic acid (deoxy-3,12-bis(TFAB)CA) . Its structure enables selective binding of carbonate ions (CO₃²⁻) through hydrogen bonding and hydrophobic interactions, making it highly effective for marine and environmental sensing applications. The ionophore exhibits Nernstian behavior (slope ≈ −30.4 mV/decade) with a detection limit of 2.8 × 10⁻⁶ M and exceptional selectivity over common interferents like Cl⁻ and SO₄²⁻ in oxygenated environments . It has been integrated into solid-state ISEs, microsensors, and redox probes for oceanic carbonate monitoring, demonstrating robustness under high-pressure deep-sea conditions and resistance to biofouling .
Preparation Methods
Synthetic Pathways and Molecular Design
Core Chemical Structure and Substituent Optimization
Carbonate Ionophore VII belongs to the class of N-alkylated cholane amides with trifluoroacetylbenzoyloxy groups. Its structure, C58H79F6NO7 (MW 1016.24 g/mol), features:
-
A cholane backbone substituted with two 4-trifluoroacetylbenzoyloxy groups at positions 3α and 12α .
-
Four n-octyl groups (C8H17) attached to the amide nitrogen, providing steric hindrance and lipophilicity .
The synthetic route involves:
-
Cholane functionalization : Esterification of 3α,12α-dihydroxy-5β-cholan-24-oic acid with 4-trifluoroacetylbenzoyl chloride under anhydrous conditions .
-
Amide formation : Reaction with n-octylamine in the presence of coupling agents (e.g., DCC/DMAP) to yield the tetra-n-octylamide derivative .
Key synthetic challenges include maintaining stereochemical integrity at the 3α and 12α positions and avoiding trifluoroacetyl group hydrolysis. Purification typically employs silica gel chromatography with ethyl acetate/hexane gradients .
Membrane Formulation and Sensor Integration
Ion-Selective Membrane Composition
This compound is incorporated into polymeric membranes for solid-state or liquid-contact ISEs. Standard formulations include:
Optimized formulation (36% PVC, 30% ionophore, 33% plasticizer, 1% ion-exchanger) achieves a Nernstian slope of −27.5 mV/decade in 10^−5–10^−3 M CO3^2− solutions .
Membrane Fabrication Protocol
-
Dissolution : 360 mg PVC, 300 mg ionophore, 330 mg bis(2-ethylhexyl) adipate, and 10 mg ion-exchanger are dissolved in 5 mL tetrahydrofuran .
-
Casting : The solution is poured into a 9 cm Petri dish, evaporated at 25°C for 24 hr, yielding ~50 μm thick membranes .
-
Sensor assembly : Membranes are mounted on screen-printed electrodes (SPEs) with poly(3-octylthiophene) as the solid contact .
Analytical Characterization and Validation
Structural Confirmation
Performance Metrics
Parameter | Value | Source |
---|---|---|
Linear range | 3.6×10^−5 – 2.5×10^−3 M | |
Response time | 30 s | |
Selectivity (log K) | Cl^−: −5.2; SO4^2−: −5.7 |
Electrochemical studies using nanopipet voltammetry reveal a carbonate transfer rate constant of 0.048 cm/s, confirming rapid ionophore-assisted transport .
Stock Concentration | Volume for 1 mM (mL) |
---|---|
1 mg/mL | 0.984 |
5 mg/mL | 4.920 |
10 mg/mL | 9.840 |
Solutions in DMSO or chloroform are stable for 1 month at −20°C or 6 months at −80°C .
Applications in Advanced Sensing Systems
Microbial Carbonate Monitoring
This compound-based nanoprobes detect CO3^2− production by Shewanella oneidensis MR-1 in bacterial media, overcoming interference from H2PO4^− and Cl^− .
Clinical Diagnostics
All-solid-state SPEs with this ionophore measure serum carbonate at pH 8.6 (adjusted with Tris-H2SO4), achieving <5% RSD in reproducibility tests .
Chemical Reactions Analysis
Structural Basis for Carbonate Binding
The ionophore features two trifluoroacetyl (TFA) groups attached to a steroidal backbone, which facilitate CO₃²⁻ recognition via hydrogen bonding or nucleophilic adduct formation (Fig. 1) .
-
Mechanism 1 : Hydrated TFA groups form hydrogen bonds with CO₃²⁻ .
-
Mechanism 2 : CO₃²⁻ attacks the electrophilic carbonyl carbon of TFA, forming a tetrahedral adduct .
Table 1: Proposed Reaction Mechanisms
Interfacial Ion-Transfer Dynamics
In nanopipet voltammetry, Carbonate Ionophore VII facilitates CO₃²⁻ transfer across water-organic interfaces via a one-step electrochemical (E) mechanism . Key parameters include:
Table 2: Kinetic Parameters for CO₃²⁻ Transfer
Parameter | Value | Method | Source |
---|---|---|---|
Rate constant () | 0.048 cm/s | Nanopipet voltammetry | |
Transfer coefficient | 0.5 | Simulation fit |
Selectivity and Interference Resistance
The ionophore exhibits high selectivity for CO₃²⁻ over common interferents (e.g., Cl⁻, SO₄²⁻) due to its hydrogen-bonding preference and steric effects . Quantitative structure-property relationship (QSPR) modeling attributes this to:
Table 3: Selectivity Coefficients
Interferent Ion | Log | Experimental Setup |
---|---|---|
Cl⁻ | -2.5 | Potentiometric membrane |
H₂PO₄⁻ | -1.8 | Bacterial culture media |
Stability and Limitations
Scientific Research Applications
Electrochemical Sensors
Ion-Selective Electrodes (ISEs) : CIVII is widely utilized in the development of ion-selective electrodes for measuring carbonate ion concentrations. These electrodes are crucial for monitoring carbon dioxide levels in various environments, including clinical settings where CO2 levels can indicate respiratory health issues. The carbonate-selective ISEs provide rapid and accurate readings, making them essential tools in healthcare diagnostics .
Performance Metrics : Recent studies have highlighted the performance of ISEs using CIVII, showcasing their sensitivity, response time, and selectivity against interfering ions. For example, a comparative analysis of different ionophores demonstrated that ISEs with CIVII exhibited superior selectivity for carbonate ions over common interferents like chloride and salicylate .
Parameter | CIVII-based ISEs | Other Ionophores |
---|---|---|
Sensitivity | High | Moderate |
Response Time | <10 seconds | Varies |
Selectivity (CO3^2- vs Cl^-) | Excellent | Poor |
Environmental Monitoring
Marine Chemistry : CIVII has been employed in marine testing to study ocean acidification and its effects on marine life. The sensors developed using CIVII can measure carbonate levels in seawater with high precision, aiding researchers in understanding the impacts of increased CO2 on marine ecosystems .
Case Study : A recent study utilized CIVII-based sensors to monitor carbonate concentrations in freshwater lakes, demonstrating a response time of less than 10 seconds and insensitivity to pressure changes. This capability allows for real-time data collection crucial for environmental assessments .
Clinical Applications
Bicarbonate Monitoring : The ability of CIVII to selectively detect bicarbonate ions is particularly valuable in clinical settings where conditions like hypercapnia (excess CO2) need to be monitored. Studies indicate that carbonate-selective ISEs can accurately measure bicarbonate levels even in the presence of salicylate, which is commonly found in medications like aspirin .
Research Findings : A study reported that CIVII-based sensors could effectively differentiate between bicarbonate and other anions, enhancing their utility in clinical diagnostics for conditions related to acid-base balance .
Advanced Research Applications
Nanoprobe Development : Recent advancements have seen the use of CIVII in nanoscale amperometric and voltammetric probes. These probes facilitate the detection of carbonate ions at extremely low concentrations, proving useful in both environmental and biological research contexts .
Mechanistic Insights : The interaction mechanisms between carbonate ions and CIVII have been thoroughly studied, revealing that the binding involves complexation processes that enhance detection sensitivity. This understanding allows for the optimization of sensor designs for specific applications .
Mechanism of Action
Carbonate ionophore VII functions by selectively binding to carbonate ions and facilitating their transport through a membrane. This selective binding is achieved through the formation of a complex between the ionophore and the carbonate ion, which is then transported across the membrane. The ionophore acts as a carrier, allowing the carbonate ion to pass through the hydrophobic environment of the membrane .
Comparison with Similar Compounds
Metal-Based Carbonate Ionophores
Recent studies have explored fluorophilic salen-derived manganese(III) complexes (Mn-1, Mn-2, Mn-3) as alternatives to tweezer-type ionophores. Key findings include:
- Selectivity : Mn-2 outperformed Mn-1 and Mn-3, achieving CO₃²⁻ selectivity 2–6 orders of magnitude higher than earlier ISEs, with reduced interference from Cl⁻ and salicylate (Sal⁻) .
- Mechanism : The Mn²⁺ center coordinates with carbonate, while the fluorophilic groups enhance lipophilicity. Mn-2’s lower steric hindrance improves binding efficiency .
- Limitations: Metal-based ionophores require precise control of the ionic site-to-ionophore molar ratio (optimal 1:4) to maintain selectivity, complicating membrane fabrication compared to Carbonate Ionophore VII .
Table 1: Performance Comparison of this compound vs. Metal-Based Complexes
Other Carbonate-Selective Ionophores
TFAP Derivatives (Ionophores I and IV)
Early TFAP-based ionophores (e.g., Ionophores I and IV) were benchmarked against this compound:
- Solubility: TFAP derivatives exhibited lower solubility in PVC membranes due to self-aggregation, whereas this compound’s cholic acid backbone ensures stable membrane integration .
- Sensitivity: Co(II)TATPP, a porphyrin-based ionophore, showed comparable potentiometric sensitivity but required complex optical calibration, limiting field applicability .
β-Cyclodextrin-TPB Ionophores
β-cyclodextrin-tetraphenylborate (β-CD:TPB) ionophores, used in modified carbon paste electrodes (CPEs), demonstrated:
- Response Slope: 125 mV/mL (electrode III) vs. 111 mV/mL for this compound-based sensors .
- Limitation: Higher ionophore quantities (10 mg vs.
Magnesium and Lithium Ionophores (Non-Carbonate Targets)
While structurally distinct, magnesium and lithium ionophores provide insights into design principles:
- Magnesium Ionophore VII (K22B5): Exhibits logP = 3.0 and selectivity for Mg²⁺ over Ca²⁺ (log K_Mg²⁺,Ca²⁻ = −2.5). Unlike this compound, it requires equimolar lipophilic salts for stability .
- Lithium Ionophore VII: Targets Li⁺ with a logP of 3.3, but its phosphonate-based structure lacks the cholic acid backbone critical for carbonate binding .
Key Advantages and Limitations of this compound
Advantages:
- Superior Selectivity : Minimal interference from Cl⁻, SO₄²⁻, and HS⁻ in oxygenated environments .
- Durability : Functions in microsensors (0.5 mm diameter) with a 60-day lifespan under deep-sea conditions .
- Versatility : Compatible with redox probes (e.g., cationic [6] helicenes) for voltammetric detection .
Limitations:
Biological Activity
Carbonate Ionophore VII is a compound that has garnered attention for its potential applications in various fields, particularly in ion-selective electrodes (ISEs) and as a chemical sensor. This article delves into its biological activity, mechanisms of action, and relevant case studies, supported by data tables and research findings.
Overview of Carbonate Ionophores
Ionophores are compounds that facilitate the transport of ions across lipid membranes, which can significantly impact biological processes. This compound specifically targets carbonate ions (), making it a useful tool in both analytical chemistry and potential therapeutic applications.
The primary mechanism by which this compound operates involves the selective binding and transport of carbonate ions. This process can alter intracellular ion concentrations, affecting various cellular functions including signaling pathways, metabolic processes, and even apoptosis.
- Ion Transport : this compound enhances the permeability of membranes to carbonate ions, which can influence cellular homeostasis.
- Cell Signaling : The modulation of ion concentrations can affect calcium signaling pathways, crucial for processes such as muscle contraction and neurotransmitter release.
- Metabolic Effects : By altering carbonate levels, this ionophore may influence metabolic pathways that rely on bicarbonate as a substrate or product.
Case Studies and Research Findings
Several studies have investigated the biological activity of this compound, highlighting its potential in various applications:
- Anticancer Activity : Research has indicated that ionophores can exhibit anticancer properties by disrupting ionic homeostasis in cancer cells. Studies have shown that ionophores like this compound can induce apoptosis in specific cancer cell lines by altering calcium signaling pathways .
- Electrochemical Sensors : As an ion-selective electrode component, this compound has been extensively studied for its ability to detect carbonate ions in biological samples. It has shown effective selectivity and sensitivity in measuring bicarbonate levels, crucial for understanding metabolic states in various diseases .
Data Tables
Q & A
Q. What is the molecular mechanism by which Carbonate Ionophore VII facilitates carbonate ion detection in potentiometric sensors?
This compound operates by forming selective 2:1 host-guest complexes with carbonate ions (CO₃²⁻) through hydrogen bonding and electrostatic interactions. The ionophore’s structure—a deoxycholic acid backbone with two trifluoroacetylbenzene (TFAB) groups—creates a binding pocket that preferentially accommodates carbonate ions over other anions. This selectivity enables Nernstian responses in ion-selective electrodes (ISEs), where the ionophore transports carbonate ions across a hydrophobic membrane, generating a measurable potential difference .
Q. How can researchers optimize sensor performance when using this compound in varying ionic strength environments (e.g., seawater vs. freshwater)?
Key methodological considerations include:
- Ionophore-to-Matrix Ratio : Adjusting the ionophore quantity (e.g., reducing from 16.6 mg to 16.0 mg) to minimize membrane crystallinity and enhance ion mobility .
- Ionic Strength Buffers : Incorporating ionic strength adjusters (e.g., NaCl for seawater simulations) to maintain consistent activity coefficients during calibration .
- Interference Testing : Systematically evaluating competing anions (e.g., Cl⁻, SO₄²⁻) using the Separate Solution Method (SSM) or Fixed Interference Method (FIM) to quantify selectivity coefficients (log) .
Q. What experimental strategies mitigate sulfide interference in carbonate sensors employing this compound?
Sulfide (HS⁻) interference is context-dependent. In oxygenated marine environments, sulfide concentrations are negligible, rendering the ionophore suitable for oceanic studies . For sulfide-rich sediments or anaerobic systems:
- Membrane Modifications : Introduce lipophilic additives (e.g., tetradodecylammonium nitrate) to reduce sulfide permeability .
- Post-Calibration Validation : Cross-validate sensor outputs with independent techniques (e.g., spectrophotometric carbonate assays) to confirm data integrity in sulfide-contaminated samples .
Q. How does the 2:1 binding stoichiometry of this compound enhance selectivity compared to 1:1 complexes?
The 2:1 binding mode (two ionophore molecules per carbonate ion) increases selectivity by maximizing complementary interactions (e.g., hydrogen bonding, steric fit) while excluding smaller anions like Cl⁻. This stoichiometry is confirmed via Job plot analyses and molecular dynamics simulations, which reveal stronger binding constants () compared to 1:1 complexes () .
Q. What are the limitations of this compound in long-term field deployments, and how can they be addressed?
- Drift in Sensitivity : Membrane fouling or ionophore leaching can occur over weeks. Mitigation strategies include:
- Temperature Dependence : Characterize response slopes across a 0–30°C range and apply temperature-compensation algorithms during data processing .
Q. How do metal-based carbonate ionophores (e.g., Mn-2 complexes) compare to this compound in terms of selectivity and detection limits?
Metal-based ionophores (e.g., Mn-2 salen derivatives) exhibit comparable selectivity for CO₃²⁻ over Cl⁻ (log) but require rigorous pH buffering due to metal-ligand dissociation at extremes. In contrast, this compound maintains stability across pH 7–9 and achieves lower detection limits ( vs. for Mn-2) due to its covalent binding mechanism .
Q. What validation protocols ensure reproducibility when synthesizing this compound for research?
- Purity Verification : Use HPLC-MS with a C18 column (acetonitrile/water gradient) to confirm >95% purity .
- Functional Testing : Validate each batch via potentiometric titrations against Na₂CO₃ standards; acceptable batches exhibit Nernstian slopes of .
Q. How can researchers reconcile contradictory data on sulfide interference reported in different studies?
Contradictions arise from differing experimental conditions (e.g., sulfide speciation, oxygen levels). Resolve discrepancies by:
Properties
IUPAC Name |
[(3R,5R,8R,9S,10S,12S,13R,14S,17R)-17-[(2R)-5-(dioctylamino)-5-oxopentan-2-yl]-10,13-dimethyl-12-[4-(2,2,2-trifluoroacetyl)benzoyl]oxy-2,3,4,5,6,7,8,9,11,12,14,15,16,17-tetradecahydro-1H-cyclopenta[a]phenanthren-3-yl] 4-(2,2,2-trifluoroacetyl)benzoate | |
---|---|---|
Details | Computed by Lexichem TK 2.7.0 (PubChem release 2021.05.07) | |
Source | PubChem | |
URL | https://pubchem.ncbi.nlm.nih.gov | |
Description | Data deposited in or computed by PubChem | |
InChI |
InChI=1S/C58H79F6NO7/c1-6-8-10-12-14-16-34-65(35-17-15-13-11-9-7-2)50(66)31-18-38(3)46-29-30-47-45-28-27-43-36-44(71-53(69)41-23-19-39(20-24-41)51(67)57(59,60)61)32-33-55(43,4)48(45)37-49(56(46,47)5)72-54(70)42-25-21-40(22-26-42)52(68)58(62,63)64/h19-26,38,43-49H,6-18,27-37H2,1-5H3/t38-,43-,44-,45+,46-,47+,48+,49+,55+,56-/m1/s1 | |
Details | Computed by InChI 1.0.6 (PubChem release 2021.05.07) | |
Source | PubChem | |
URL | https://pubchem.ncbi.nlm.nih.gov | |
Description | Data deposited in or computed by PubChem | |
InChI Key |
PNOQSKXIBWVYCX-PNZPGYLPSA-N | |
Details | Computed by InChI 1.0.6 (PubChem release 2021.05.07) | |
Source | PubChem | |
URL | https://pubchem.ncbi.nlm.nih.gov | |
Description | Data deposited in or computed by PubChem | |
Canonical SMILES |
CCCCCCCCN(CCCCCCCC)C(=O)CCC(C)C1CCC2C1(C(CC3C2CCC4C3(CCC(C4)OC(=O)C5=CC=C(C=C5)C(=O)C(F)(F)F)C)OC(=O)C6=CC=C(C=C6)C(=O)C(F)(F)F)C | |
Details | Computed by OEChem 2.3.0 (PubChem release 2021.05.07) | |
Source | PubChem | |
URL | https://pubchem.ncbi.nlm.nih.gov | |
Description | Data deposited in or computed by PubChem | |
Isomeric SMILES |
CCCCCCCCN(CCCCCCCC)C(=O)CC[C@@H](C)[C@H]1CC[C@@H]2[C@@]1([C@H](C[C@H]3[C@H]2CC[C@H]4[C@@]3(CC[C@H](C4)OC(=O)C5=CC=C(C=C5)C(=O)C(F)(F)F)C)OC(=O)C6=CC=C(C=C6)C(=O)C(F)(F)F)C | |
Details | Computed by OEChem 2.3.0 (PubChem release 2021.05.07) | |
Source | PubChem | |
URL | https://pubchem.ncbi.nlm.nih.gov | |
Description | Data deposited in or computed by PubChem | |
Molecular Formula |
C58H79F6NO7 | |
Details | Computed by PubChem 2.1 (PubChem release 2021.05.07) | |
Source | PubChem | |
URL | https://pubchem.ncbi.nlm.nih.gov | |
Description | Data deposited in or computed by PubChem | |
Molecular Weight |
1016.2 g/mol | |
Details | Computed by PubChem 2.1 (PubChem release 2021.05.07) | |
Source | PubChem | |
URL | https://pubchem.ncbi.nlm.nih.gov | |
Description | Data deposited in or computed by PubChem | |
Disclaimer and Information on In-Vitro Research Products
Please be aware that all articles and product information presented on BenchChem are intended solely for informational purposes. The products available for purchase on BenchChem are specifically designed for in-vitro studies, which are conducted outside of living organisms. In-vitro studies, derived from the Latin term "in glass," involve experiments performed in controlled laboratory settings using cells or tissues. It is important to note that these products are not categorized as medicines or drugs, and they have not received approval from the FDA for the prevention, treatment, or cure of any medical condition, ailment, or disease. We must emphasize that any form of bodily introduction of these products into humans or animals is strictly prohibited by law. It is essential to adhere to these guidelines to ensure compliance with legal and ethical standards in research and experimentation.