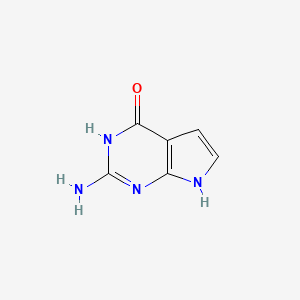
7-Deazaguanine
Overview
Description
7-Deazaguanine is a modified purine analog characterized by the replacement of the nitrogen atom at position 7 of guanine with a carbon atom (C–H) . This structural alteration eliminates a major groove hydrogen bond donor, modifies electronic properties, and enhances resistance to enzymatic degradation . Key features include:
- Chemical Properties: Exhibits distinct tautomeric forms and increased hydrophobicity, facilitating stable interactions with metal ions .
- Biological Roles: Found in tRNA (e.g., queuosine, archaeosine) to enhance translational accuracy and tRNA stability . In DNA, it serves as a defense mechanism in bacterial restriction-modification systems and phage anti-restriction strategies .
Preparation Methods
Biosynthetic Pathways in Bacterial Systems
The Dpd Gene Cluster and PreQ0 Biosynthesis
The bacterial dpd gene cluster encodes the enzymatic machinery for incorporating 7-deazaguanine derivatives into DNA. In Salmonella enterica serovar Montevideo, the pathway begins with the conversion of GTP to 7-cyano-7-deazaguanine (preQ0), a shared precursor for tRNA queuosine (Q) modifications and DNA this compound derivatives . The initial steps involve GTP cyclohydrolase I (GCHI), which produces dihydroneopterin triphosphate (H2NTP), followed by QueD and QueE enzymes that catalyze the formation of 7-carboxy-7-deazaguanine (CDG) . QueC then adenylates CDG to generate 7-amido-7-deazaguanine (ADG), which undergoes dehydration to yield preQ0 . This intermediate is critical for both tRNA and DNA modification pathways, creating potential substrate competition in vivo .
Enzymatic Insertion of preQ0 into DNA
The DpdA and DpdB proteins are essential for inserting preQ0 into bacterial DNA. DpdA, a homolog of tRNA-guanine transglycosylase (TGT), catalyzes a transglycosylation reaction that replaces guanine with preQ0 in DNA . Structural studies reveal that DpdA binds DNA through a conserved active site, with residues Tyr106 and Glu142 critical for base excision and preQ0 incorporation . DpdB, an ATPase, facilitates this process by hydrolyzing ATP to stabilize DNA-protein interactions or modulate DpdA activity . In vitro reconstitution experiments demonstrate that a system containing 5 μM DpdA, 5 μM DpdB, 20 μM preQ0, and 10 mM ATP achieves complete modification of pUC19 DNA within 60 minutes at 37°C .
Table 1: Key Enzymes in Bacterial this compound Biosynthesis
Conversion to 2'-Deoxy-7-Amido-7-Deazaguanosine (dADG)
Following preQ0 insertion, DpdC hydrolyzes the nitrile group of DNA-embedded preQ0 to form dADG . This amidase activity requires no additional cofactors beyond water, with kinetic studies showing a kcat of 2.3 ± 0.1 min⁻¹ and KM of 15 ± 2 μM for preQ0-modified DNA . The dual-functionality of the dpd cluster—modifying DNA while providing restriction-enzyme resistance—highlights the evolutionary interplay between bacterial defense systems and nucleotide metabolism .
Enzymatic Modification in Phage DNA
Viral Utilization of this compound Derivatives
Archaeal and bacteriophage genomes employ this compound modifications to evade host restriction systems. Over 180 phages encode homologs of DpdA or QueC, with 60% targeting pathogenic hosts like Escherichia coli and Salmonella . For example, Escherichia phage CAjan uses DpdA to insert dPreQ1 (2'-deoxy-7-aminomethyl-7-deazaguanine) into its DNA, conferring resistance to 12 out of 15 tested restriction enzymes .
Pathway Intermediates in Phage Genomes
Phage DNA modifications include three intermediates of the this compound pathway:
-
dADG : Formed via phage-encoded amidases analogous to DpdC .
-
dPreQ1 : A reduced derivative involving aminomethylation, unique to viral systems .
Mass spectrometry of Enterobacteria phage 9g DNA revealed dADG at 0.8–1.2 modifications per 1,000 bases, compared to 0.2–0.4 for dPreQ0 . This stoichiometry suggests phages prioritize later pathway intermediates to maximize restriction evasion.
Chemical Synthesis of this compound Derivatives
Boc-Protected Synthesis of Pyrrolo[2,3-d]Pyrimidinones
A 2009 route enables the synthesis of this compound nucleosides via tert-butoxycarbonyl (Boc) protection strategies . The protocol involves:
-
Cyclization : Treating 4-chloro-5-iodopyrrolo[2,3-d]pyrimidine with NaN3 to form an azide intermediate.
-
Staudinger Reaction : Converting the azide to a phosphazene using triphenylphosphine.
-
Hydrolysis : Acidic cleavage yields 2-amino-7-deazaguanine derivatives .
Table 2: Key Reactions in Chemical Synthesis
Step | Reagents | Conditions | Yield |
---|---|---|---|
Cyclization | NaN3, DMF | 80°C, 12 h | 78% |
Staudinger | PPh3, THF | RT, 2 h | 92% |
Hydrolysis | HCl (6M) | 100°C, 4 h | 85% |
Advantages and Limitations
Chemical synthesis achieves milligram-scale production with purity >95% (HPLC) . However, it lacks the regioselectivity of enzymatic methods, often requiring chromatographic separation of N7 vs. N9 isomers . In contrast, DpdA-based systems exclusively modify DNA at guanine residues, avoiding isomer contamination .
Comparative Analysis of Preparation Methods
Efficiency and Scalability
-
Biosynthesis : Requires 60 minutes for complete DNA modification but depends on recombinant protein expression.
-
Phage Systems : Native phage enzymes modify DNA during replication, enabling high-throughput modification in infected hosts .
-
Chemical Synthesis : Suitable for analog development (e.g., 5-methyl-7-deazaguanine) but limited by multi-step purification .
Applications in Biotechnology
Chemical Reactions Analysis
Chemical Modifications and Their Functions
The presence of 7-deazaguanine derivatives in DNA serves critical functions, particularly in protecting phage genomes from restriction enzymes. The following modifications have been identified:
-
2′-Deoxy-7-amido-7-deazaguanine (dADG) : This derivative plays a role in evading host restriction systems.
-
2′-Deoxy-7-cyano-7-deazaguanine (dPreQ0) : Acts as an intermediate in both tRNA modification pathways and DNA protection mechanisms.
-
2′-Deoxy-7-(methylamino)methyl-7-deazaguanine (mdPreQ1) : Newly identified derivative that enhances genomic stability.
These modifications are crucial for the survival of certain bacterial strains and phages, allowing them to resist degradation by host nucleases .
Enzymatic Mechanisms
Recent studies have elucidated the roles of various enzymes involved in the incorporation of this compound into DNA:
-
DpdA : A transglycosylase that facilitates the insertion of preQ0 into DNA through a unique mechanism involving ATP hydrolysis.
-
DpdB : Exhibits ATPase activity essential for the transglycosylation process, highlighting an unexpected interaction between energy metabolism and nucleobase modification.
The structural analysis of these enzymes has revealed critical active site residues necessary for their catalytic functions, providing insights into their mechanisms .
Protective Functions Against Restriction Enzymes
The incorporation of this compound derivatives into viral genomes has been shown to confer resistance against bacterial restriction-modification systems. For instance:
-
Phages encoding DpdA exhibit enhanced survival rates when infecting bacterial hosts due to the protective nature of modified bases like dADG and dPreQ0.
This discovery underscores the evolutionary arms race between phages and bacteria, where modifications such as those involving this compound play pivotal roles in genetic stability and survival .
Data Tables
Scientific Research Applications
Viral Genomic Protection
One of the primary applications of 7-deazaguanine is its role in protecting viral genomic DNA from host restriction enzymes. Research has shown that bacteriophages utilize this compound modifications to evade bacterial defense mechanisms. For instance, a study identified multiple derivatives of this compound in phage DNA that help these viruses resist host restriction-modification (RM) systems .
Key Findings:
- Modification Mechanism: The insertion of 2′-deoxy-7-deazaguanine is facilitated by enzymes such as DpdA, which acts as a guanine transglycosylase .
- Diversity of Modifications: Eight derivatives have been cataloged, including previously unknown forms that enhance the resilience of phage genomes against host defenses .
Synthetic Biology
In synthetic biology, this compound derivatives have been employed to create nucleic acid constructs with enhanced stability and reduced susceptibility to nucleases. These properties make them valuable for developing novel biotechnological applications such as gene editing and therapeutic delivery systems.
Applications in Synthetic Biology:
- tRNA Modifications: Certain derivatives like queuosine are involved in minimizing translational errors and stabilizing tRNA molecules .
- Gene Editing Tools: The unique properties of this compound allow for innovative approaches in genome editing technologies, such as CRISPR/Cas9 systems, where they can be used to modify guide RNAs or target sequences for improved specificity and efficiency .
Epigenetic Regulation
Recent studies have highlighted the role of this compound in epigenetic modifications within bacterial systems. These modifications can influence gene expression patterns and cellular responses to environmental changes.
Research Insights:
- Bacterial Defense Mechanisms: The presence of this compound derivatives in bacterial DNA serves as a defense mechanism against phage attacks, showcasing an evolutionary arms race between bacteria and viruses .
- Functional Implications: The incorporation of these modifications into DNA has implications for understanding bacterial adaptation and resilience .
Data Tables
Case Studies
- Bacteriophage CAjan :
- CRISPR/Cas9 Systems :
Mechanism of Action
The mechanism of action of 7-deazaguanine involves its incorporation into DNA and tRNA, where it replaces guanine. This modification alters the structure and function of the nucleic acids, affecting processes such as translation and replication. The molecular targets include tRNA-guanine transglycosylase enzymes, which catalyze the exchange of guanine for this compound .
Comparison with Similar Compounds
Structural and Functional Comparison
The following table summarizes key 7-deazaguanine derivatives and related compounds:
Key Distinctions
- Hydrogen Bonding: this compound lacks the N7 hydrogen bond donor, reducing major groove protein interactions compared to guanine . Derivatives like preQ₁ restore cationic interactions via aminomethyl groups .
- Enzymatic Synthesis :
- Functional Specialization :
DNA Protection Mechanisms
- This compound derivatives in phage DNA (e.g., dADG, dPreQ₀) reduce restriction enzyme binding by 10–100-fold, enhancing viral persistence .
- Escherichia phage 9g mutants lacking this compound show 90% reduced fitness against BREX and RADAR host defenses .
Thermodynamic and Structural Impacts
- DNA Stability: this compound incorporation decreases duplex stability (ΔTm = −3°C per modification) due to disrupted hydration . However, 7-aminomethyl derivatives restore stability via cationic interactions .
- Electron Transfer: this compound facilitates ultrafast electron transfer (5–75 ps) in DNA, independent of donor-acceptor distance, enabling applications in biosensors .
Enzymatic and Evolutionary Insights
- Substrate Plasticity: QueF enzymes, initially misannotated as GTP cyclohydrolases, instead reduce preQ₀ to preQ₁ using NADPH .
- Horizontal Gene Transfer: Genomic islands (e.g., dpdA-K cluster in Salmonella) encode this compound modification systems, suggesting evolutionary adaptation for foreign DNA defense .
Data Tables
Table 1. Enzymatic Pathways for this compound Derivatives
Biological Activity
7-Deazaguanine (7DG) is a modified nucleobase derived from guanine, notable for its unique structural and functional properties. It has been identified in various biological systems, particularly in bacteriophages and certain bacterial species, where it plays critical roles in protecting genomic DNA from host restriction enzymes. This article explores the biological activity of this compound, focusing on its mechanisms of action, derivatives, and implications in molecular biology.
Structural Characteristics
This compound is characterized by the substitution of the nitrogen atom at position 7 of the guanine base with a carbon atom. This modification alters the hydrogen bonding and steric properties of the base, influencing its interactions within nucleic acids. The structural variation leads to several derivatives, including:
Derivative | Chemical Structure | Function |
---|---|---|
2′-deoxy-7-deazaguanine (dDG) | ![dDG structure] | Protects viral DNA from restriction enzymes |
2′-deoxy-7-amido-7-deazaguanine (dADG) | ![dADG structure] | Modifies DNA to evade host defenses |
2′-deoxy-7-carboxy-7-deazaguanine (dCDG) | ![dCDG structure] | Involved in DNA stability and function |
Protection Against Restriction Enzymes
One of the primary biological activities of this compound is its role in protecting viral genomic DNA from bacterial restriction-modification (RM) systems. Bacteriophages have evolved mechanisms to incorporate this compound into their DNA, allowing them to evade degradation by host restriction enzymes. For instance, studies have shown that phages such as Enterobacteria phage 9g utilize this compound modifications to replace guanine bases in their genomes, thereby enhancing resistance against host defenses .
Modification Pathways
The insertion of this compound into DNA is facilitated by specific enzymes known as guanine transglycosylases. The DpdA family of proteins plays a crucial role in this process. Recent research has identified multiple subfamilies of DpdA that exhibit varying affinities for different derivatives of this compound, highlighting the evolutionary adaptation of phages to optimize their genomic integrity .
Phage Genomes
A comprehensive analysis of phage genomes revealed that over 180 phages encode for enzymes involved in the synthesis and incorporation of this compound derivatives. Notably, the Escherichia phage CAjan was found to replace up to 32% of its guanine bases with preQ0 (a derivative), showcasing a significant modification that enhances its survival against bacterial defenses .
Bacterial Systems
In bacteria, the presence of this compound modifications has been linked to RM systems encoded by dpd genes located within genomic clusters. These systems are essential for bacterial survival against phage infections and highlight the ongoing arms race between bacteria and their viral counterparts .
Research Findings
Recent studies have provided insights into the biosynthetic pathways that generate various this compound derivatives. Key enzymes involved include:
- DpdL : Catalyzes the decarboxylation of carboxy-derivatives.
- DpdN : Responsible for formyltransferase activities.
- DpdM : Functions as a methyltransferase, contributing to further modifications .
These findings underscore the complexity and adaptability of molecular mechanisms involving this compound across different organisms.
Properties
IUPAC Name |
2-amino-3,7-dihydropyrrolo[2,3-d]pyrimidin-4-one | |
---|---|---|
Source | PubChem | |
URL | https://pubchem.ncbi.nlm.nih.gov | |
Description | Data deposited in or computed by PubChem | |
InChI |
InChI=1S/C6H6N4O/c7-6-9-4-3(1-2-8-4)5(11)10-6/h1-2H,(H4,7,8,9,10,11) | |
Source | PubChem | |
URL | https://pubchem.ncbi.nlm.nih.gov | |
Description | Data deposited in or computed by PubChem | |
InChI Key |
OLAFFPNXVJANFR-UHFFFAOYSA-N | |
Source | PubChem | |
URL | https://pubchem.ncbi.nlm.nih.gov | |
Description | Data deposited in or computed by PubChem | |
Canonical SMILES |
C1=CNC2=C1C(=O)NC(=N2)N | |
Source | PubChem | |
URL | https://pubchem.ncbi.nlm.nih.gov | |
Description | Data deposited in or computed by PubChem | |
Molecular Formula |
C6H6N4O | |
Source | PubChem | |
URL | https://pubchem.ncbi.nlm.nih.gov | |
Description | Data deposited in or computed by PubChem | |
DSSTOX Substance ID |
DTXSID30223745 | |
Record name | 7-Deazaguanine | |
Source | EPA DSSTox | |
URL | https://comptox.epa.gov/dashboard/DTXSID30223745 | |
Description | DSSTox provides a high quality public chemistry resource for supporting improved predictive toxicology. | |
Molecular Weight |
150.14 g/mol | |
Source | PubChem | |
URL | https://pubchem.ncbi.nlm.nih.gov | |
Description | Data deposited in or computed by PubChem | |
CAS No. |
7355-55-7 | |
Record name | 7-Deazaguanine | |
Source | ChemIDplus | |
URL | https://pubchem.ncbi.nlm.nih.gov/substance/?source=chemidplus&sourceid=0007355557 | |
Description | ChemIDplus is a free, web search system that provides access to the structure and nomenclature authority files used for the identification of chemical substances cited in National Library of Medicine (NLM) databases, including the TOXNET system. | |
Record name | 7355-55-7 | |
Source | DTP/NCI | |
URL | https://dtp.cancer.gov/dtpstandard/servlet/dwindex?searchtype=NSC&outputformat=html&searchlist=62498 | |
Description | The NCI Development Therapeutics Program (DTP) provides services and resources to the academic and private-sector research communities worldwide to facilitate the discovery and development of new cancer therapeutic agents. | |
Explanation | Unless otherwise indicated, all text within NCI products is free of copyright and may be reused without our permission. Credit the National Cancer Institute as the source. | |
Record name | 7-Deazaguanine | |
Source | EPA DSSTox | |
URL | https://comptox.epa.gov/dashboard/DTXSID30223745 | |
Description | DSSTox provides a high quality public chemistry resource for supporting improved predictive toxicology. | |
Record name | 7-DEAZAGUANINE | |
Source | FDA Global Substance Registration System (GSRS) | |
URL | https://gsrs.ncats.nih.gov/ginas/app/beta/substances/GPL8T5ZO3M | |
Description | The FDA Global Substance Registration System (GSRS) enables the efficient and accurate exchange of information on what substances are in regulated products. Instead of relying on names, which vary across regulatory domains, countries, and regions, the GSRS knowledge base makes it possible for substances to be defined by standardized, scientific descriptions. | |
Explanation | Unless otherwise noted, the contents of the FDA website (www.fda.gov), both text and graphics, are not copyrighted. They are in the public domain and may be republished, reprinted and otherwise used freely by anyone without the need to obtain permission from FDA. Credit to the U.S. Food and Drug Administration as the source is appreciated but not required. | |
Synthesis routes and methods I
Procedure details
Synthesis routes and methods II
Procedure details
Retrosynthesis Analysis
AI-Powered Synthesis Planning: Our tool employs the Template_relevance Pistachio, Template_relevance Bkms_metabolic, Template_relevance Pistachio_ringbreaker, Template_relevance Reaxys, Template_relevance Reaxys_biocatalysis model, leveraging a vast database of chemical reactions to predict feasible synthetic routes.
One-Step Synthesis Focus: Specifically designed for one-step synthesis, it provides concise and direct routes for your target compounds, streamlining the synthesis process.
Accurate Predictions: Utilizing the extensive PISTACHIO, BKMS_METABOLIC, PISTACHIO_RINGBREAKER, REAXYS, REAXYS_BIOCATALYSIS database, our tool offers high-accuracy predictions, reflecting the latest in chemical research and data.
Strategy Settings
Precursor scoring | Relevance Heuristic |
---|---|
Min. plausibility | 0.01 |
Model | Template_relevance |
Template Set | Pistachio/Bkms_metabolic/Pistachio_ringbreaker/Reaxys/Reaxys_biocatalysis |
Top-N result to add to graph | 6 |
Feasible Synthetic Routes
Disclaimer and Information on In-Vitro Research Products
Please be aware that all articles and product information presented on BenchChem are intended solely for informational purposes. The products available for purchase on BenchChem are specifically designed for in-vitro studies, which are conducted outside of living organisms. In-vitro studies, derived from the Latin term "in glass," involve experiments performed in controlled laboratory settings using cells or tissues. It is important to note that these products are not categorized as medicines or drugs, and they have not received approval from the FDA for the prevention, treatment, or cure of any medical condition, ailment, or disease. We must emphasize that any form of bodily introduction of these products into humans or animals is strictly prohibited by law. It is essential to adhere to these guidelines to ensure compliance with legal and ethical standards in research and experimentation.