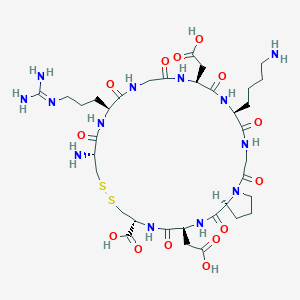
iRGD peptide
Overview
Description
The iRGD peptide (sequence: CRGDK/RGPD/EC) is a disulfide-bonded cyclic peptide that combines integrin-targeting and neuropilin-1 (NRP-1)-binding properties . Its mechanism involves a two-step process:
Integrin Binding: The RGD motif binds to αvβ3/αvβ5 integrins overexpressed on tumor vasculature and cancer cells .
Proteolytic Activation: Enzymatic cleavage exposes a C-terminal CendR motif (R/KXXR/K), which binds NRP-1, triggering tissue penetration and cellular internalization via macropinocytosis .
iRGD enhances tumor delivery of co-administered drugs or nanoparticles by up to 10-fold compared to conventional RGD peptides . It also exhibits intrinsic anti-metastatic activity by inhibiting β1 integrin-mediated adhesion and inducing chemorepulsion through NRP-1 .
Biological Activity
The iRGD peptide (CRGDKGPDC) is a cyclic peptide that has garnered significant attention in cancer research due to its unique biological activities, particularly its ability to enhance drug delivery to tumors and inhibit metastasis. This article explores the mechanisms by which iRGD operates, its applications in cancer therapy, and relevant research findings.
The biological activity of iRGD is primarily attributed to its dual-targeting capability, which allows it to bind to both integrins and neuropilin-1 (NRP-1) receptors that are overexpressed in tumor vasculature. The mechanism can be summarized in several key steps:
- Binding to Integrins : The RGD motif within iRGD binds to αv integrins on tumor cells and endothelial cells, facilitating initial attachment.
- Proteolytic Cleavage : After binding, iRGD is cleaved by proteases, exposing the CRGDK fragment that specifically binds to NRP-1.
- Tumor Penetration : The interaction with NRP-1 triggers internalization and enhances the permeability of tumor blood vessels, allowing for deeper penetration into the tumor stroma.
This multistep process significantly improves the delivery of therapeutic agents, making iRGD a promising candidate for enhancing chemotherapy efficacy.
Research Findings
Numerous studies have demonstrated the effectiveness of iRGD in various cancer models. Below are some notable findings:
Case Studies
- Pancreatic Cancer Model :
- Lung Cancer Study :
- Colorectal Cancer :
Advantages of Using iRGD
The application of this compound presents several advantages in cancer treatment:
- Enhanced Drug Delivery : By improving drug penetration into tumors, iRGD can potentially overcome the limitations associated with traditional chemotherapeutics that struggle to reach their targets effectively.
- Reduced Side Effects : Targeted delivery minimizes exposure of healthy tissues to cytotoxic drugs, thereby reducing side effects.
- Versatility : iRGD can be used with various therapeutic agents, including chemotherapeutics and immunotherapies, enhancing their effectiveness .
Scientific Research Applications
Tumor Targeting and Drug Delivery
Mechanism of Action:
- The iRGD peptide binds initially to αv integrins prevalent in tumor blood vessels, triggering proteolytic cleavage that exposes the CRGDK fragment.
- This fragment subsequently binds to neuropilin-1, promoting deeper penetration into the tumor microenvironment.
Applications in Drug Delivery:
- Nanoparticle Conjugation: iRGD-modified nanoparticles have shown improved tumor absorption when administered intravenously. For instance, Wang et al. demonstrated that iRGD-modified iron oxide nanoworms enhanced MRI signal intensity in tumors compared to traditional methods .
- Chemotherapeutic Delivery: The co-administration of iRGD with chemotherapeutic agents has been shown to significantly increase their efficacy. Studies indicate that iRGD enhances the distribution of drugs like doxorubicin throughout tumor tissues, leading to better therapeutic outcomes and reduced side effects .
Imaging Applications
Enhanced Imaging Techniques:
- iRGD has been utilized to improve imaging modalities for cancer detection. The peptide's ability to selectively penetrate tumors allows for more accurate imaging of malignant tissues.
- Sugahara et al. demonstrated that iRGD-linked imaging agents could effectively target tumors in vivo, providing clear visualization through MRI techniques .
Case Studies:
- Near-Infrared Fluorescence Imaging: Ye et al. synthesized near-infrared fluorescence-labeled iRGD peptides that successfully localized tumors in animal models, showcasing the peptide's potential for real-time imaging applications .
Therapeutic Strategies
Combination Therapies:
- The integration of iRGD into various therapeutic frameworks has shown promise in enhancing treatment efficacy:
- Oncolytic Viruses: Puig-Saus et al. incorporated iRGD into oncolytic adenoviruses, resulting in improved penetration and antitumor effects in xenograft models .
- Immunotherapy Enhancement: Recent studies indicate that using iRGD can amplify the effects of immune checkpoint inhibitors by improving drug delivery to tumor sites .
Innovative Approaches:
- Researchers have explored modifying existing peptides with iRGD to enhance their stability and solubility while maintaining their targeting capabilities. For example, the ATAP-iRGD modification has shown improved selective delivery to tumors with significant reductions in tumor size observed in animal studies .
Q & A
Basic Research Questions
Q. What is the molecular mechanism underlying iRGD-mediated tumor targeting and tissue penetration?
iRGD binds to integrin αvβ3 via its RGD motif, triggering proteolytic cleavage to expose a CendR motif (R/KXXR/K) that interacts with neuropilin-1 (NRP-1). This dual binding activates endocytic transport, enabling deep tumor penetration . Methodologically, validate this mechanism using:
- Integrin αvβ3/NRP-1 knockout models to assess receptor dependency.
- Mass spectrometry (e.g., MALDI-TOF) to confirm peptide cleavage and degradation products .
- Competitive binding assays with unlabeled RGD peptides (e.g., c(RGDfK) ) to evaluate selectivity.
Q. What experimental models are optimal for initial validation of iRGD’s tumor-targeting efficacy?
Use orthotopic or patient-derived xenograft (PDX) models with confirmed αvβ3/NRP-1 expression. Key steps:
- Immunohistochemistry to quantify receptor density in tumor vasculature .
- Optical/PET imaging (e.g., using 64Cu-DOTA or 18F-BEM-labeled iRGD analogs) to track tumor accumulation .
- Pharmacokinetic studies comparing free drugs versus iRGD-conjugated formulations .
Q. How does iRGD compare to conventional cyclic RGD peptides in integrin binding and tumor penetration?
iRGD exhibits lower αvβ3 affinity than c(RGDfK) but superior tumor penetration due to NRP-1-mediated transport. Experimental approaches:
- Surface plasmon resonance (SPR) to measure binding kinetics .
- 3D tumor spheroids to assess penetration depth via confocal microscopy .
- In vivo biodistribution studies using dual-labeled probes (e.g., fluorescent/PET) .
Advanced Research Questions
Q. How can iRGD be structurally modified to enhance isoform selectivity (e.g., αvβ3 vs. αvβ5)?
- Alanine scanning mutagenesis to identify residues critical for integrin isoform binding .
- Conformational analysis via NMR or molecular dynamics simulations to optimize peptide rigidity .
- Peptide libraries with non-natural amino acids (e.g., D-amino acids) to improve stability and selectivity .
- In vitro selectivity assays using recombinant integrin isoforms .
Q. What methodologies address discrepancies in iRGD efficacy across tumor models (e.g., reproducibility challenges)?
Contradictory findings (e.g., failed co-administration efficacy ) require:
- Preclinical validation of peptide activity via SPR or cell adhesion assays prior to in vivo use .
- Biomarker stratification (e.g., NRP-1 expression levels in tumors) using RNA-seq or flow cytometry .
- Standardized dosing protocols (e.g., molar ratio of iRGD to therapeutic payload) .
Q. How do co-administration strategies influence iRGD’s pharmacokinetics and therapeutic synergy?
Co-injecting iRGD with chemotherapeutics enhances passive targeting (EPR effect) and active transport. Key considerations:
- Temporal sequencing : Administer iRGD 30–60 minutes before therapeutics to prime tumor vasculature .
- Dose optimization : Use molar ratios of 1:10 (iRGD:drug) to avoid receptor saturation .
- Toxicity profiling : Monitor off-target effects (e.g., renal clearance) via serum creatinine/BUN assays .
Q. What analytical techniques quantify iRGD’s tumor penetration and drug delivery efficiency?
- Multiphoton microscopy for real-time visualization of peptide extravasation .
- LC-MS/MS to measure intratumoral drug concentrations .
- Microdialysis to sample interstitial fluid and quantify unbound drug levels .
Q. Methodological Tables
Table 1. Key Receptors and Assays for iRGD Characterization
Receptor | Assay Type | Readout | Reference |
---|---|---|---|
Integrin αvβ3 | Competitive SPR | KD values vs. c(RGDfK) | |
NRP-1 | Fluorescence polarization | Binding affinity (nM) | |
Tumor tissue | MALDI-TOF imaging | Spatial distribution |
Table 2. Strategies to Improve iRGD Reproducibility
Challenge | Solution | Reference |
---|---|---|
Variable NRP-1 levels | Pre-screen tumors via IHC/RNA-seq | |
Batch-to-batch variability | Request peptide content analysis (HPLC) | |
Off-target toxicity | Use renal/hepatic function tests |
Comparison with Similar Compounds
Conventional Cyclic RGD Peptides (e.g., c(RGDfK))
Key Findings :
- c(RGDfK) exhibits higher αvβ3 binding affinity but lacks enzymatic activation for tissue penetration .
- iRGD outperforms c(RGDfK) in delivering payloads to hypoxic tumor regions .
Non-CendR Control Peptides (e.g., CRGDC, iRGE)
- Fails to penetrate tumors or inhibit metastasis .
- iRGE: A variant of iRGD lacking the RGD motif. Binds NRP-1 but cannot initiate integrin-mediated targeting, resulting in 60% lower tumor accumulation .
Mechanistic Insight :
The CendR motif is critical for NRP-1-mediated internalization. CRGDC and iRGE highlight the necessity of dual targeting (integrin + NRP-1) for effective delivery .
Sema3A-Derived Peptide
A 22-amino acid peptide from Sema3A’s C-terminus shares iRGD’s NRP-1-binding CendR motif. Both induce cytoskeletal collapse and chemorepulsion . However, Sema3A-derived peptides lack integrin-targeting RGD sequences, limiting their tumor specificity .
LyP-1 and CK3 Peptides
- LyP-1 : A tumor-penetrating peptide targeting p32/GRP76. While effective in lymphatic tumors, it lacks integrin-binding capability and has narrower application than iRGD .
Engineered iRGD Analogs
Modified iRGD analogs (e.g., DOTA-Cys-iRGD) enable dual-modality imaging (optical/PET) and show 3–5× higher tumor retention than non-targeted probes . These modifications retain the CendR motif’s functionality while expanding diagnostic applications .
Preparation Methods
Solid-Phase Peptide Synthesis (SPPS) of Linear iRGD Precursors
The foundation of iRGD production lies in solid-phase peptide synthesis (SPPS), which enables precise control over amino acid sequence assembly. Studies uniformly employ Fmoc (fluorenylmethyloxycarbonyl) chemistry due to its compatibility with acid-labile side-chain protections . The linear peptide chain is constructed on Rink amide resin, which provides a stable amide linkage for subsequent cyclization.
Resin Activation and Amino Acid Coupling
The MBHA Rink amide resin is pre-treated with 20% piperidine in dimethylformamide (DMF) to remove the Fmoc group from the initial amino acid . Each subsequent residue—Cys(Acm), Arg(Pbf), Gly, Asp(OtBu), Lys(Boc), Pro, and others—is coupled using HATU (1-[bis(dimethylamino)methylene]-1H-1,2,3-triazolo[4,5-b]pyridinium 3-oxid hexafluorophosphate) as the activating agent in the presence of N-methyl morpholine (NMM) . Coupling efficiencies exceeding 98% are achieved by optimizing molar ratios (3:1 amino acid-to-resin excess) and reaction times (20–30 minutes per residue) .
Terminal Modifications
N-terminal acetylation and C-terminal amidation are critical for enhancing proteolytic stability. For example, Source acetylates the N-terminal cysteine using acetic anhydride, while C-terminal amidation is achieved by using Rink amide resin, which inherently generates a carboxamide upon cleavage . These modifications reduce enzymatic degradation without altering integrin-binding affinity .
Cyclization Strategies for Disulfide Bridge Formation
Cyclization via a disulfide bond between cysteine residues is essential for iRGD’s tumor-targeting activity. Two primary methods dominate the literature: thallium trifluoroacetate-mediated oxidation and air oxidation .
Thallium Trifluoroacetate (Tl(OOCCF₃)₃)
This reagent facilitates rapid disulfide bond formation on solid support. After linear synthesis, the resin-bound peptide is treated with 0.1 M Tl(OOCCF₃)₃ in DMF for 2–4 hours, achieving >95% cyclization efficiency . The reaction mechanism involves thallium(III) oxidizing cysteine thiols to form a cyclic dimer, which is stabilized by the Acm (acetamidomethyl) protecting groups . Post-cyclization, the Acm groups are retained to prevent over-oxidation.
Air Oxidation
Alternative protocols use diluted aqueous solutions (pH 8.0) under atmospheric oxygen, though this method requires extended reaction times (24–48 hours) and yields lower cyclization rates (~70%) . It is less favored due to risks of side reactions, such as methionine oxidation or aspartimide formation.
Cleavage and Purification Protocols
Resin Cleavage
Peptides are cleaved from the resin using trifluoroacetic acid (TFA)-based cocktails. A typical mixture of TFA:thioanisole:triisopropylsilane:water (90:5:2.5:2.5) simultaneously removes acid-labile protecting groups (e.g., OtBu from Asp, Boc from Lys, Pbf from Arg) . The reaction is quenched with cold diethyl ether, precipitating the crude peptide as a white powder.
High-Performance Liquid Chromatography (HPLC)
Purification employs reverse-phase HPLC with C18 columns and gradients of acetonitrile/water containing 0.1% TFA. For iRGD, a gradient from 10% to 80% acetonitrile over 30 minutes achieves baseline separation of cyclic and linear forms . Semi-preparative columns (250 × 10 mm) operated at 4 mL/min yield >98% purity, as confirmed by analytical HPLC .
Purification Parameter | Conditions | Source |
---|---|---|
Column | XBridge BEH130 C18 (10 μm, 250 × 10 mm) | |
Gradient | 10–80% acetonitrile in 30 min | |
Flow Rate | 4 mL/min | |
Detection | UV at 230 nm |
Structural Confirmation and Quality Control
Mass Spectrometry
Matrix-assisted laser desorption ionization time-of-flight (MALDI-TOF) and electrospray ionization (ESI) mass spectrometry validate molecular weights. For iRGD, the observed m/z of 1551.2295 ([M+H]⁺) matches the theoretical m/z of 1551.7201 . Disulfide bond integrity is confirmed by treating the peptide with tris(2-carboxyethyl)phosphine (TCEP), which reduces the cyclic form to a linear peptide with a +2 Da mass shift .
Analytical HPLC
Peptide homogeneity is assessed using C18 analytical columns (4.6 × 250 mm, 5 μm). The cyclic iRGD elutes at 14.12 min, while its reduced form elutes later (14.53 min) due to increased hydrophilicity .
Modified iRGD Analogs and Conjugates
Fluorescent Probes
Source details the synthesis of Cy5.5-labeled iRGD by conjugating Cy5.5-NHS ester to the N-terminal cysteine. The reaction achieves 39% yield after HPLC purification, with MALDI-TOF confirming a mass of 2202.9662 ([M+H]⁺) .
Antibody-Peptide Conjugates
A glycoengineering approach links iRGD to anti-PD-1 antibodies via fucosyltransferase-mediated glycosylation. The LacNAc-conju platform attaches GDP-Fuc-iRGD to Fc-glycans, achieving a peptide-to-antibody ratio of 1.88:1 . ESI-MS confirms the conjugate mass of 148,833 Da .
Challenges and Optimization Strategies
Cyclization Efficiency
Thallium-based methods, while efficient, pose toxicity concerns. Alternatives like iodine oxidation in methanol:water (1:1) are explored but yield suboptimal cyclization (~80%) .
Scalability
SPPS is limited by resin loading capacity (typically 0.2–0.5 mmol/g). Large-scale production may require segment condensation or native chemical ligation, though these methods remain underdeveloped for iRGD.
Properties
IUPAC Name |
(6S,9S,15S,18R,23R,26S,29S)-18-amino-6-(4-aminobutyl)-9,26-bis(carboxymethyl)-15-[3-(diaminomethylideneamino)propyl]-2,5,8,11,14,17,25,28-octaoxo-20,21-dithia-1,4,7,10,13,16,24,27-octazabicyclo[27.3.0]dotriacontane-23-carboxylic acid | |
---|---|---|
Source | PubChem | |
URL | https://pubchem.ncbi.nlm.nih.gov | |
Description | Data deposited in or computed by PubChem | |
InChI |
InChI=1S/C35H57N13O14S2/c36-8-2-1-5-18-30(57)42-14-25(50)48-10-4-7-23(48)33(60)46-21(12-27(53)54)32(59)47-22(34(61)62)16-64-63-15-17(37)28(55)44-19(6-3-9-40-35(38)39)29(56)41-13-24(49)43-20(11-26(51)52)31(58)45-18/h17-23H,1-16,36-37H2,(H,41,56)(H,42,57)(H,43,49)(H,44,55)(H,45,58)(H,46,60)(H,47,59)(H,51,52)(H,53,54)(H,61,62)(H4,38,39,40)/t17-,18-,19-,20-,21-,22-,23-/m0/s1 | |
Source | PubChem | |
URL | https://pubchem.ncbi.nlm.nih.gov | |
Description | Data deposited in or computed by PubChem | |
InChI Key |
YHTTWXCDIRTOQX-FQJIPJFPSA-N | |
Source | PubChem | |
URL | https://pubchem.ncbi.nlm.nih.gov | |
Description | Data deposited in or computed by PubChem | |
Canonical SMILES |
C1CC2C(=O)NC(C(=O)NC(CSSCC(C(=O)NC(C(=O)NCC(=O)NC(C(=O)NC(C(=O)NCC(=O)N2C1)CCCCN)CC(=O)O)CCCN=C(N)N)N)C(=O)O)CC(=O)O | |
Source | PubChem | |
URL | https://pubchem.ncbi.nlm.nih.gov | |
Description | Data deposited in or computed by PubChem | |
Isomeric SMILES |
C1C[C@H]2C(=O)N[C@H](C(=O)N[C@@H](CSSC[C@@H](C(=O)N[C@H](C(=O)NCC(=O)N[C@H](C(=O)N[C@H](C(=O)NCC(=O)N2C1)CCCCN)CC(=O)O)CCCN=C(N)N)N)C(=O)O)CC(=O)O | |
Source | PubChem | |
URL | https://pubchem.ncbi.nlm.nih.gov | |
Description | Data deposited in or computed by PubChem | |
Molecular Formula |
C35H57N13O14S2 | |
Source | PubChem | |
URL | https://pubchem.ncbi.nlm.nih.gov | |
Description | Data deposited in or computed by PubChem | |
Molecular Weight |
948.0 g/mol | |
Source | PubChem | |
URL | https://pubchem.ncbi.nlm.nih.gov | |
Description | Data deposited in or computed by PubChem | |
CAS No. |
1392278-76-0 | |
Record name | IRGD | |
Source | ChemIDplus | |
URL | https://pubchem.ncbi.nlm.nih.gov/substance/?source=chemidplus&sourceid=1392278760 | |
Description | ChemIDplus is a free, web search system that provides access to the structure and nomenclature authority files used for the identification of chemical substances cited in National Library of Medicine (NLM) databases, including the TOXNET system. | |
Retrosynthesis Analysis
AI-Powered Synthesis Planning: Our tool employs the Template_relevance Pistachio, Template_relevance Bkms_metabolic, Template_relevance Pistachio_ringbreaker, Template_relevance Reaxys, Template_relevance Reaxys_biocatalysis model, leveraging a vast database of chemical reactions to predict feasible synthetic routes.
One-Step Synthesis Focus: Specifically designed for one-step synthesis, it provides concise and direct routes for your target compounds, streamlining the synthesis process.
Accurate Predictions: Utilizing the extensive PISTACHIO, BKMS_METABOLIC, PISTACHIO_RINGBREAKER, REAXYS, REAXYS_BIOCATALYSIS database, our tool offers high-accuracy predictions, reflecting the latest in chemical research and data.
Strategy Settings
Precursor scoring | Relevance Heuristic |
---|---|
Min. plausibility | 0.01 |
Model | Template_relevance |
Template Set | Pistachio/Bkms_metabolic/Pistachio_ringbreaker/Reaxys/Reaxys_biocatalysis |
Top-N result to add to graph | 6 |
Feasible Synthetic Routes
Disclaimer and Information on In-Vitro Research Products
Please be aware that all articles and product information presented on BenchChem are intended solely for informational purposes. The products available for purchase on BenchChem are specifically designed for in-vitro studies, which are conducted outside of living organisms. In-vitro studies, derived from the Latin term "in glass," involve experiments performed in controlled laboratory settings using cells or tissues. It is important to note that these products are not categorized as medicines or drugs, and they have not received approval from the FDA for the prevention, treatment, or cure of any medical condition, ailment, or disease. We must emphasize that any form of bodily introduction of these products into humans or animals is strictly prohibited by law. It is essential to adhere to these guidelines to ensure compliance with legal and ethical standards in research and experimentation.