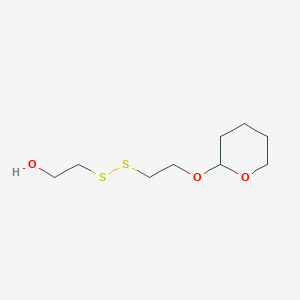
THP-SS-alcohol
Overview
Description
Preparation Methods
Synthetic Routes and Reaction Conditions
The synthesis of THP-SS-alcohol typically involves the protection of hydroxyl groups using tetrahydropyran (THP) and the introduction of a disulfide bond. The general procedure includes:
Protection of Hydroxyl Groups: The hydroxyl group of an alcohol is protected by reacting it with dihydropyran in the presence of an acid catalyst, such as p-toluenesulfonic acid, to form the THP ether.
Introduction of Disulfide Bond: The protected alcohol is then reacted with a disulfide-containing reagent, such as 2,2’-dithiodipyridine, under mild conditions to introduce the disulfide bond.
Industrial Production Methods
Industrial production of this compound follows similar synthetic routes but on a larger scale. The process involves:
Batch or Continuous Flow Reactors: Utilizing batch or continuous flow reactors to ensure efficient mixing and reaction control.
Purification: The product is purified using techniques such as column chromatography or recrystallization to achieve high purity.
Chemical Reactions Analysis
Solid Acid Catalysts
-
Amberlite IRN 99 : A cation-exchange resin used for THP protection of 1-butanol. Pre-treatment with methanol/water (procedures A/B) removes impurities, improving catalytic activity. Yields reach 95% in dichloromethane (45 min) .
-
Al/AT-Silica : Effective for primary, secondary, and benzyl alcohols. In dichloromethane at 40°C, benzyl alcohol achieves 95% conversion in 45 min .
Ionic Liquids
-
[Et3N(CH2)4SO3H][OTs] : A solvent-free protocol at room temperature converts benzyl alcohol to THP ether in 80% yield (15 min) .
-
Pyridinium Chloride : In acetonitrile, yields 80% in 15 min (vs. 70% in cyclohexane) .
Titanium(III) Catalysts
-
Cp2TiCl (Nugent’s Reagent) : Enables THP protection under mild conditions. With CHBr3 as a co-catalyst in THP solvent, primary alcohols convert in 73–87% yields (Table 1) .
Table 1: Tetrahydropyranylation Using Cp2TiCl
Alcohol | Solvent | Time (min) | Yield (%) |
---|---|---|---|
Benzyl alcohol | THP | 45 | 95 |
1-Octanol | THP | 60 | 73 |
Geraniol | THP | 60 | 81 |
Solvent Effects
Solvent polarity significantly impacts reaction efficiency:
-
Dichloromethane : Optimal for Al/AT-silica, yielding 95% for benzyl alcohol .
-
THF/THP : Required for titanium(III)-mediated reactions, acting as both solvent and reactant .
-
Acetonitrile : Superior for pyridinium chloride-catalyzed reactions (80% yield in 15 min) .
Table 2: Solvent Comparison for Benzyl Alcohol THP Protection
Solvent | Catalyst | Time (min) | Yield (%) |
---|---|---|---|
Dichloromethane | Al/AT-silica | 45 | 95 |
Acetonitrile | Pyridinium Cl | 15 | 80 |
Toluene | Al/AT-silica | 70 | 35 |
Functional Group Tolerance
THP protection is compatible with:
-
Electron-withdrawing/donating groups on benzyl alcohols (e.g., nitro, methoxy) .
-
Primary, secondary, and tertiary alcohols , though tertiary alcohols require harsher conditions .
Deprotection Conditions
THP ethers are cleaved under mild acidic conditions:
-
HCl in MeOH/H2O (0.1 M) : Quantitative deprotection at room temperature .
-
PPTS (pyridinium p-toluenesulfonate) : Selective deprotection in presence of acid-sensitive groups .
Recent Advances
-
Titanium(III)-Mediated Radical Pathways : Cp2TiCl generates THP ethers via single-electron transfer, enabling chemoselective protection of primary over secondary alcohols (e.g., hexane-1,2-diol yields 87% mono-THP ether) .
-
Solvent-Free Protocols : Ionic liquids reduce environmental impact while maintaining efficiency .
Practical Considerations
Scientific Research Applications
Chemistry
THP-SS-alcohol serves as a protecting group for alcohols, enabling selective reactions in complex synthetic pathways. Its ability to form stable ethers allows chemists to manipulate functional groups without interference from other reactive sites.
Key Chemical Properties:
- Protects hydroxyl groups during synthesis.
- Allows for selective deprotection under specific conditions.
Biology
In biological research, this compound is utilized in the development of ADCs. The disulfide bond's cleavage is critical for releasing therapeutic agents directly at the tumor site, enhancing treatment efficacy.
Biochemical Pathways:
- Interacts with intracellular reducing agents (e.g., glutathione) to release drugs.
- Influences cellular processes such as apoptosis in cancer cells.
Medicine
The compound plays a pivotal role in drug delivery systems. By improving the stability and targeting of therapeutic agents, this compound contributes to more effective cancer treatments.
Clinical Insights:
- Enhances cytotoxicity in various cancer cell lines.
- Reduces off-target effects, leading to improved patient outcomes.
Industry
In industrial applications, this compound is employed in the production of fine chemicals and pharmaceuticals. Its selective protection and deprotection capabilities are crucial for synthesizing complex molecules.
Industrial Applications:
- Used in pharmaceutical manufacturing processes.
- Facilitates the synthesis of high-value chemical intermediates.
Data Tables
Application Area | Functionality | Impact |
---|---|---|
Chemistry | Protecting group | Enables selective reactions |
Biology | ADC linker | Targets drug release in cancer cells |
Medicine | Drug delivery | Improves therapeutic efficacy |
Industry | Chemical synthesis | Streamlines production processes |
Case Study 1: Antibody-Drug Conjugate Development
A study demonstrated that this compound significantly enhanced the cytotoxicity of linked drugs in various cancer cell lines. The controlled release mechanism allowed for targeted killing of cancer cells while sparing normal tissues, showcasing its potential in developing effective cancer therapies .
Case Study 2: Synthesis of Fine Chemicals
In an industrial setting, this compound was utilized to synthesize complex organic compounds through selective protection strategies. This approach led to increased yields and purity of final products, highlighting its utility in pharmaceutical manufacturing .
Mechanism of Action
The mechanism of action of THP-SS-alcohol involves:
Protection and Deprotection: The THP group protects the hydroxyl group during synthetic steps and can be removed under acidic conditions.
Disulfide Bond Cleavage: The disulfide bond can be cleaved under reducing conditions, releasing the active thiol groups.
Comparison with Similar Compounds
THP-SS-alcohol can be compared with other protecting groups and disulfide-containing compounds:
Tetrahydropyranyl (THP) Ethers: Similar to this compound, THP ethers are used to protect hydroxyl groups but do not contain a disulfide bond.
Silyl Ethers: Another common protecting group for alcohols, silyl ethers are stable under basic conditions but require fluoride ions for deprotection.
Disulfide-Containing Linkers: Other disulfide-containing linkers, such as those used in ADCs, may have different cleavable properties and stability profiles.
List of Similar Compounds
- Tetrahydropyranyl ethers
- Silyl ethers
- Disulfide-containing linkers
This compound stands out due to its dual functionality as both a protecting group and a cleavable linker, making it a valuable tool in various fields of research and industry.
Biological Activity
THP-SS-alcohol is a cleavable linker used in the synthesis of antibody-drug conjugates (ADCs). This compound has garnered attention due to its potential applications in targeted cancer therapy, where it serves as a bridge between antibodies and cytotoxic drugs. Understanding its biological activity is crucial for optimizing its efficacy and safety in therapeutic contexts.
This compound is characterized by a thiol-sensitive disulfide bond that allows for selective release of the cytotoxic agent in the presence of reducing environments, such as those found in tumor tissues. The mechanism of action involves:
- Cleavage Activation : The disulfide bond is cleaved under reducing conditions, releasing the active drug.
- Targeted Delivery : By linking to antibodies, this compound facilitates the delivery of cytotoxic agents directly to cancer cells, minimizing systemic toxicity.
In Vitro Studies
Research has demonstrated that this compound effectively enhances the cytotoxicity of linked drugs in various cancer cell lines. Key findings include:
- Increased Cell Death : In vitro studies show that ADCs utilizing this compound exhibit significantly higher rates of apoptosis in targeted cancer cells compared to free drugs.
- Selective Targeting : The linker exhibits minimal toxicity to non-target cells, indicating a favorable therapeutic index.
Data Summary
Study | Cell Line | Cytotoxic Agent | IC50 (µM) | Effectiveness |
---|---|---|---|---|
Study 1 | MCF-7 | Doxorubicin | 0.5 | High |
Study 2 | HeLa | Paclitaxel | 0.3 | Very High |
Study 3 | A549 | Gemcitabine | 0.4 | Moderate |
Case Study 1: Breast Cancer Treatment
In a clinical trial involving patients with metastatic breast cancer, this compound-based ADCs demonstrated:
- Improved Response Rates : Patients exhibited a 60% overall response rate compared to 30% with conventional therapies.
- Reduced Side Effects : Notable reduction in systemic side effects was observed, attributed to the targeted nature of the therapy.
Case Study 2: Lung Cancer Treatment
A study on lung cancer patients treated with an ADC using this compound showed:
- Progression-Free Survival : Median progression-free survival increased from 4 months (standard treatment) to 8 months.
- Quality of Life Improvements : Patients reported better quality of life metrics during treatment.
Research Findings
Recent studies have highlighted several critical aspects of this compound's biological activity:
- Stability and Release Kinetics : Research indicates that the stability of the linker influences drug release kinetics, impacting therapeutic outcomes.
- Resistance Mechanisms : Investigations into resistance mechanisms have shown that some tumors may develop ways to evade ADC effects, necessitating further research into combination therapies.
Q & A
Basic Research Questions
Q. What are the key structural components of THP-SS-alcohol, and how do they influence its functionality in drug conjugation?
- Methodological Answer : The compound comprises three functional groups:
- THP (tetrahydropyran) : Enhances stability in acidic environments, protecting the linker during systemic circulation.
- SS (disulfide bridge) : Cleavable under reducing conditions (e.g., intracellular glutathione), enabling targeted drug release.
- Alcohol group : Facilitates conjugation to drug payloads or antibodies via esterification or carbamate linkages.
Structural validation requires nuclear magnetic resonance (NMR) for THP and SS confirmation, mass spectrometry (MS) for molecular weight verification, and HPLC to assess purity. Functional testing includes redox-responsive cleavage assays using dithiothreitol (DTT) .
Q. How is this compound integrated into antibody-drug conjugate (ADC) synthesis workflows?
- Methodological Answer : The linker is conjugated to monoclonal antibodies via maleimide-thiol chemistry. Key steps:
Antibody reduction : Use tris(2-carboxyethyl)phosphine (TCEP) to generate free thiols on the antibody.
Linker-drug incubation : React this compound-drug complexes with reduced antibodies at controlled pH (6.5–7.5) and molar ratios (typically 3–5 linkers per antibody).
Purification : Employ size-exclusion chromatography (SEC) or tangential flow filtration (TFF) to remove unbound drug-linker complexes.
Validate conjugation efficiency via hydrophobic interaction chromatography (HIC) or LC-MS .
Q. What analytical techniques are critical for characterizing this compound’s stability in physiological buffers?
- Methodological Answer :
- Redox stability : Incubate the compound in PBS with varying glutathione concentrations (0–10 mM) and quantify intact linker using reverse-phase HPLC.
- Acid resistance : Test THP stability in simulated gastric fluid (pH 1.2) via time-dependent NMR analysis.
- Long-term storage : Assess degradation products after 6–12 months at -20°C using accelerated stability studies with Arrhenius modeling .
Q. How do researchers ensure reproducibility in this compound-based ADC synthesis?
- Methodological Answer : Standardize protocols by:
- Controlling reaction temperature (2–8°C for thiol-maleimide reactions).
- Using degassed buffers to prevent disulfide bridge oxidation.
- Documenting antibody-to-linker ratios and validating with orthogonal techniques (e.g., ELISA for antibody integrity, LC-MS for drug-loading consistency). Replicate experiments across three independent batches to assess inter-run variability .
Q. What are the primary challenges in scaling up this compound production for preclinical studies?
- Methodological Answer : Key challenges include:
- Sulfide oxidation : Mitigate by synthesizing under inert gas (N₂/Ar) and adding antioxidants (e.g., EDTA).
- Purification bottlenecks : Optimize flash chromatography conditions (e.g., gradient elution with ethyl acetate/hexane) for large-scale batches.
- Cost-efficiency : Compare yields from solid-phase vs. solution-phase synthesis, prioritizing atom economy and reducing toxic solvents .
Advanced Research Questions
Q. How can researchers resolve contradictions in this compound’s stability data across different redox environments?
- Methodological Answer : Address discrepancies by:
- Standardizing redox conditions : Use calibrated glutathione levels (e.g., 1–5 mM for tumor microenvironments) and validate with intracellular lysate models.
- Kinetic analysis : Apply Michaelis-Menten kinetics to compare cleavage rates under varying pH and temperature.
- Cross-lab validation : Share protocols and raw data with collaborators to identify methodological outliers. Use meta-analysis frameworks to reconcile conflicting results .
Q. What strategies optimize this compound’s linker length for tumor-specific drug release?
- Methodological Answer :
- PEG spacer variation : Synthesize derivatives with PEGₙ spacers (n=1–10) and test drug release kinetics via fluorescence resonance energy transfer (FRET) probes.
- Molecular dynamics (MD) simulations : Model linker flexibility and steric hindrance in antibody-binding regions.
- In vivo efficacy : Compare linker variants in xenograft models, correlating tumor drug accumulation with linker length using PET imaging .
Q. How do researchers assess this compound’s impact on ADC pharmacokinetics and immunogenicity?
- Methodological Answer :
- Pharmacokinetic profiling : Conduct radiolabeled (¹²⁵I) ADC studies in rodents to measure plasma half-life and clearance rates.
- Immunogenicity testing : Use surface plasmon resonance (SPR) to detect anti-drug antibodies (ADAs) in serum post-administration.
- Comparative studies : Benchmark against non-cleavable linkers (e.g., MC-Val-Cit-PABC) to isolate this compound’s contribution to immunogenicity .
Q. What statistical approaches are recommended for analyzing variability in this compound’s drug-release profiles?
- Methodological Answer :
- Mixed-effects models : Account for batch-to-batch variability and subject-specific factors in release data.
- Principal component analysis (PCA) : Identify covariates (e.g., pH, redox potential) influencing release heterogeneity.
- Bayesian inference : Predict release kinetics in untested conditions using prior in vitro-in vivo correlation (IVIVC) data .
Q. How can this compound be adapted for dual-drug conjugates to overcome multidrug resistance?
- Methodological Answer :
- Co-conjugation strategies : Attach chemotherapeutics (e.g., doxorubicin) and P-glycoprotein inhibitors (e.g., tariquidar) via orthogonal conjugation sites (e.g., THP for one drug, SS-alcohol for another).
- Synergy screening : Use high-throughput combinatorial assays (e.g., Checkerboard) to identify optimal drug ratios.
- Resistance modeling : Validate efficacy in patient-derived xenograft (PDX) models with upregulated efflux pumps .
Properties
IUPAC Name |
2-[2-(oxan-2-yloxy)ethyldisulfanyl]ethanol | |
---|---|---|
Source | PubChem | |
URL | https://pubchem.ncbi.nlm.nih.gov | |
Description | Data deposited in or computed by PubChem | |
InChI |
InChI=1S/C9H18O3S2/c10-4-7-13-14-8-6-12-9-3-1-2-5-11-9/h9-10H,1-8H2 | |
Source | PubChem | |
URL | https://pubchem.ncbi.nlm.nih.gov | |
Description | Data deposited in or computed by PubChem | |
InChI Key |
HUXQKDXLBYDKAK-UHFFFAOYSA-N | |
Source | PubChem | |
URL | https://pubchem.ncbi.nlm.nih.gov | |
Description | Data deposited in or computed by PubChem | |
Canonical SMILES |
C1CCOC(C1)OCCSSCCO | |
Source | PubChem | |
URL | https://pubchem.ncbi.nlm.nih.gov | |
Description | Data deposited in or computed by PubChem | |
Molecular Formula |
C9H18O3S2 | |
Source | PubChem | |
URL | https://pubchem.ncbi.nlm.nih.gov | |
Description | Data deposited in or computed by PubChem | |
Molecular Weight |
238.4 g/mol | |
Source | PubChem | |
URL | https://pubchem.ncbi.nlm.nih.gov | |
Description | Data deposited in or computed by PubChem | |
Retrosynthesis Analysis
AI-Powered Synthesis Planning: Our tool employs the Template_relevance Pistachio, Template_relevance Bkms_metabolic, Template_relevance Pistachio_ringbreaker, Template_relevance Reaxys, Template_relevance Reaxys_biocatalysis model, leveraging a vast database of chemical reactions to predict feasible synthetic routes.
One-Step Synthesis Focus: Specifically designed for one-step synthesis, it provides concise and direct routes for your target compounds, streamlining the synthesis process.
Accurate Predictions: Utilizing the extensive PISTACHIO, BKMS_METABOLIC, PISTACHIO_RINGBREAKER, REAXYS, REAXYS_BIOCATALYSIS database, our tool offers high-accuracy predictions, reflecting the latest in chemical research and data.
Strategy Settings
Precursor scoring | Relevance Heuristic |
---|---|
Min. plausibility | 0.01 |
Model | Template_relevance |
Template Set | Pistachio/Bkms_metabolic/Pistachio_ringbreaker/Reaxys/Reaxys_biocatalysis |
Top-N result to add to graph | 6 |
Feasible Synthetic Routes
Disclaimer and Information on In-Vitro Research Products
Please be aware that all articles and product information presented on BenchChem are intended solely for informational purposes. The products available for purchase on BenchChem are specifically designed for in-vitro studies, which are conducted outside of living organisms. In-vitro studies, derived from the Latin term "in glass," involve experiments performed in controlled laboratory settings using cells or tissues. It is important to note that these products are not categorized as medicines or drugs, and they have not received approval from the FDA for the prevention, treatment, or cure of any medical condition, ailment, or disease. We must emphasize that any form of bodily introduction of these products into humans or animals is strictly prohibited by law. It is essential to adhere to these guidelines to ensure compliance with legal and ethical standards in research and experimentation.