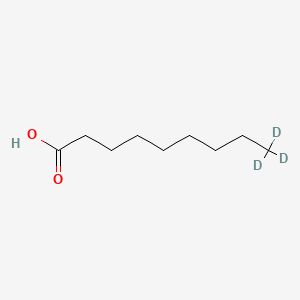
Nonanoic-9,9,9-D3 acid
Overview
Description
Nonanoic acid (C₉H₁₈O₂), also known as pelargonic acid, is a saturated medium-chain fatty acid (C9:0) naturally produced by geraniums (Pelargonium spp.) and other plants. It is widely studied for its herbicidal properties, role in atmospheric chemistry, and biological activities, including histone deacetylase (HDAC) inhibition and modulation of tumor phenotypes . Nonanoic-9,9,9-D3 acid is a deuterated isotopologue where three hydrogen atoms at the terminal methyl group (C9) are replaced with deuterium. This modification is typically used in metabolic tracing and spectroscopic studies to track biochemical pathways without significantly altering chemical reactivity .
Preparation Methods
Synthetic Routes and Reaction Conditions: The synthesis of Nonanoic-9,9,9-D3 acid typically involves the deuteration of nonanoic acid. One common method is the catalytic exchange of hydrogen atoms with deuterium using a deuterium gas atmosphere and a suitable catalyst, such as palladium on carbon (Pd/C). The reaction is carried out under high pressure and elevated temperatures to ensure complete deuteration.
Industrial Production Methods: Industrial production of this compound follows similar principles but on a larger scale. The process involves the use of continuous flow reactors to maintain consistent reaction conditions and improve yield. The deuterium gas is supplied in large quantities, and the reaction is monitored to ensure the desired level of deuteration is achieved.
Chemical Reactions Analysis
Types of Reactions: Nonanoic-9,9,9-D3 acid undergoes similar chemical reactions as nonanoic acid, including:
Oxidation: It can be oxidized to form nonanoic acid derivatives.
Reduction: It can be reduced to form nonanol.
Substitution: It can participate in substitution reactions where the carboxyl group is replaced by other functional groups.
Common Reagents and Conditions:
Oxidation: Common oxidizing agents include potassium permanganate (KMnO₄) and chromium trioxide (CrO₃).
Reduction: Reducing agents such as lithium aluminum hydride (LiAlH₄) or sodium borohydride (NaBH₄) are used.
Substitution: Reagents like thionyl chloride (SOCl₂) can be used to convert the carboxyl group to an acyl chloride, which can then undergo further substitution reactions.
Major Products:
Oxidation: Nonanoic acid derivatives.
Reduction: Nonanol.
Substitution: Various substituted nonanoic acid derivatives.
Scientific Research Applications
Metabolic Studies
Nonanoic-9,9,9-D3 acid is utilized in metabolic tracing studies due to its isotopic labeling. This property allows researchers to investigate metabolic pathways and the fate of fatty acids in biological systems. For instance, studies have shown that using deuterated fatty acids can improve the accuracy of tracing lipid metabolism in various organisms.
Case Study:
A study involving the use of this compound demonstrated its effectiveness in tracing lipid metabolism in mice. The results indicated that this compound could be effectively incorporated into cellular membranes, allowing researchers to assess lipid turnover rates under different dietary conditions.
Biocatalysis
Recent advancements in bioprocess engineering have explored the use of recombinant microorganisms for the production of fatty acid derivatives. This compound has been produced using engineered strains of Corynebacterium glutamicum, which express specific enzymes for biotransformation.
Data Table: Production Conditions for this compound
Parameter | Optimal Condition |
---|---|
Cultivation Temperature | 15 °C |
Reaction Temperature | 30 °C |
Detergent Concentration | 0.05 g/L Tween 80 |
pH | 7.5 |
Conversion Yield | 75.6% (mol/mol) |
This method highlights the potential for sustainable production of isotopically labeled fatty acids using microbial systems.
Drug Development
This compound serves as a valuable tool in drug development processes. Its isotopic labeling aids in pharmacokinetic studies by allowing precise tracking of drug absorption and metabolism in vivo.
Case Study:
In a pharmacological study focused on anti-inflammatory compounds derived from fatty acids, this compound was used to trace the metabolic pathways of potential drug candidates. The findings revealed insights into how modifications to fatty acid structures can enhance therapeutic efficacy while minimizing side effects.
Soil and Water Studies
The degradation and behavior of this compound in various environmental matrices are crucial for understanding its ecological impact. Research has indicated that deuterated fatty acids can provide insights into soil microbial activity and pollutant degradation.
Data Table: Environmental Behavior Studies
Parameter | Observed Effect |
---|---|
Soil Type | Sandy vs. Clay |
Degradation Rate | Higher in sandy soils |
Microbial Activity | Enhanced with D-labeled compounds |
These studies are essential for assessing the environmental fate of fatty acids used in agricultural applications or as surfactants.
Mechanism of Action
The mechanism of action of Nonanoic-9,9,9-D3 acid is similar to that of nonanoic acid, but the presence of deuterium atoms can influence reaction rates and pathways due to the kinetic isotope effect. This effect arises because deuterium has a greater mass than hydrogen, leading to differences in bond vibrational frequencies and reaction kinetics.
Comparison with Similar Compounds
Medium-Chain Fatty Acids (C8–C11)
Nonanoic acid’s phytotoxicity and membrane interactions are highly dependent on carbon chain length. Evidence from Fukuda et al. (2004) indicates that fatty acids with C9–C11 chains exhibit the highest herbicidal activity compared to shorter (C8) or longer (C12+) chains . This is attributed to their optimal balance of hydrophobicity and solubility, enabling efficient plasma membrane penetration and destabilization .
Table 1. Comparative Properties of Medium-Chain Fatty Acids
Natural Phytotoxins: Sarmentine and Sorgoleone
Nonanoic acid is less effective at partitioning into lipid bilayers compared to structurally distinct phytotoxins like sarmentine (a piperideine alkaloid) and sorgoleone (a lipid benzoquinone). Isothermal titration calorimetry (ITC) studies rank their membrane affinities as: sorgoleone > sarmentine > nonanoic acid . However, nonanoic acid’s rapid phytotoxic action arises from direct membrane disruption rather than mitochondrial inhibition (a mechanism observed in sorgoleone) .
Table 2. Membrane Affinity and Phytotoxic Mechanisms
Structural Analogs in HDAC Inhibition
Nonanoic acid shares structural similarity with valproic acid, a known HDAC inhibitor and mood stabilizer.
Table 3. HDAC Inhibitors and Structural Analogs
Functional Analogs in Industrial and Environmental Chemistry
- IR3535 (Ethyl Butylacetylaminopropionate): In repellent formulations, nonanoic acid forms pseudo-azeotropic mixtures with IR3535, altering evaporation rates and enhancing persistence .
- Oleic Acid Derivatives: Nonanoic acid is a major product of oleic acid ozonolysis in marine surface microlayers, contributing to atmospheric organic aerosols .
- Octanoic Acid: Both acids are volatile components in foods (e.g., kombucha, meat), but nonanoic acid is more resistant to thermal decomposition during cooking .
Table 4. Stability and Environmental Roles
Key Research Findings and Contradictions
- Phytotoxicity vs. Membrane Affinity: While nonanoic acid has lower membrane affinity than sorgoleone , its rapid herbicidal action stems from simpler membrane disruption rather than complex biochemical inhibition .
- Tumor Phenotype Modulation: Nonanoic acid induces dose-dependent metabolic changes in small intestinal neuroendocrine tumors (SI-NETs) via OR51E1 receptor activation, but high concentrations (>300 µM) cause nonspecific toxicity .
- Surface Chemistry: At the air/water interface, nonanoic acid’s pKa shifts by ~1 unit due to interfacial packing, enhancing its persistence in marine aerosols .
Biological Activity
Nonanoic-9,9,9-D3 acid, a derivative of nonanoic acid, has garnered attention for its biological activities, particularly in antimicrobial and antifungal applications. This article synthesizes current research findings regarding its biological effects, mechanisms of action, and potential applications.
- Molecular Formula : C₉H₁₈O₂
- Molecular Weight : 158.238 g/mol
- CAS Number : 134646-27-8
- Melting Point : 9 °C
- Boiling Point : 268-269 °C
- Density : 0.906 g/mL at 25 °C
Antimicrobial Activity
Research indicates that this compound exhibits significant antimicrobial properties:
- Bacterial Inhibition : It has been shown to reduce bacterial translocation and enhance antibacterial activity. Specifically, it increases the secretion of porcine β-defensins (pBD-1 and pBD-2), which play a critical role in the innate immune response against pathogens .
- Fungal Activity : The compound demonstrates antifungal capabilities against various pathogens. Studies have indicated its effectiveness in inhibiting mycelial growth and spore germination in plant pathogenic fungi such as M. roreri and C. perniciosa in a concentration-dependent manner .
The mechanisms underlying the biological activities of this compound are multifaceted:
- Histone Deacetylase Inhibition : Nonanoic acid is known to upregulate endogenous host defense peptides through histone deacetylase inhibition, enhancing the intestinal epithelial immunological barrier function . This suggests a potential epigenetic mechanism by which the compound exerts its effects on immune responses.
- Cell Membrane Interaction : As a saturated fatty acid, Nonanoic acid may interact with cell membranes, altering their permeability and disrupting the integrity of microbial cells, which contributes to its antimicrobial effects .
Case Studies and Research Findings
Several studies have explored the biological activity of nonanoic acids:
Q & A
Basic Research Questions
Q. How is Nonanoic-9,9,9-D3 acid synthesized, and what methods ensure isotopic purity in deuterated derivatives?
this compound is synthesized via selective deuteration at the terminal methyl group (CD₃). Isotopic purity (>99 atom% D) is achieved through controlled exchange reactions or catalytic deuteration, followed by purification via distillation or chromatography. Characterization involves nuclear magnetic resonance (NMR) spectroscopy to confirm deuterium placement and mass spectrometry to quantify isotopic enrichment. Stability during storage requires inert atmospheres and low temperatures to prevent proton exchange .
Q. What key physical properties of this compound are critical for experimental design?
Critical properties include:
- Melting point : ~285 K (pure acid) .
- Boiling point : ~526 K at atmospheric pressure .
- Solubility : Limited aqueous solubility (0.01–1 mM at pH 2–12), enhanced in organic solvents (e.g., ethanol, hexane). Supercritical CO₂ solubility ranges from 0.14 kg·m⁻³ (333 K, 10 MPa) to 25.39 kg·m⁻³ (313 K, 30 MPa) . These properties inform solvent selection, temperature control, and pressure conditions for extraction or reaction setups .
Q. How can gas chromatography (GC) be optimized for quantifying nonanoic acid in complex matrices?
GC optimization involves:
- Internal standards : Use deuterated analogs (e.g., Nonanoic-2,2-D2 acid) to correct for matrix effects .
- Response factors : Calibrate using external standards (0.1–15 mM) and calculate sensitivity ratios between target analytes and reference compounds (e.g., lauric acid) .
- Column selection : Polar stationary phases (e.g., DB-WAX) improve separation of carboxylic acids from co-eluting compounds .
Advanced Research Questions
Q. How do surface and bulk acid dissociation constants (pKa) of nonanoic acid differ, and what integrated methodologies resolve this?
The bulk pKa of nonanoic acid is 4.8 ± 0.1 (measured via NMR at 0.1–0.9 mM), while the surface pKa at the air/water interface is 5.9 ± 0.6 (determined via free energy perturbation (FEP) molecular dynamics (MD) simulations). The 1.1-unit shift arises from reduced solvation at the interface. Combined surface tension measurements, infrared reflection absorption spectroscopy (IRRAS), and MD simulations are required to decouple bulk-surface partitioning from dissociation effects .
Q. What role do molecular dynamics (MD) simulations play in elucidating nonanoic acid’s interfacial behavior?
MD simulations (CHARMM36 force field, NAMD 2.13) model monolayer stability, headgroup orientation, and ion interactions. For example:
- Protonated (HA) vs. deprotonated (A⁻) forms : HA forms stable monolayers (30 Ų/lipid), while A⁻ exhibits reduced surface activity due to electrostatic repulsion.
- Salt effects : Na⁺ stabilizes A⁻ at interfaces via ion pairing, increasing surface adsorption by ~5× in 0.5 M NaCl. Alchemical FEP calculations quantify free energy differences (ΔΔG) between bulk and surface dissociation states .
Q. How does ionic strength (e.g., NaCl) perturb nonanoic acid’s surface adsorption and bulk dissociation equilibria?
NaCl (0.5 M) decreases bulk pKa to 4.6 ± 0.1 (vs. 4.8 in pure water) by stabilizing deprotonation via Na⁺ coordination. At the surface:
- HA adsorption : Increases marginally (6–66% higher surface pressure at pH 2).
- A⁻ adsorption : Increases 5× due to Na⁺-COO⁻ interactions, validated by IRRAS peak integration . Thermodynamic models (e.g., van der Waals adsorption isotherms) must account for salt-induced activity coefficient changes .
Q. What thermodynamic models describe nonanoic acid’s partitioning between bulk and surface phases under variable pH?
A four-species model (HAbulk, A⁻bulk, HAsurface, A⁻surface) integrates:
- Bulk-surface partitioning : Fitted via surface pressure-concentration curves at pH 2.1 and 11.5.
- Acid dissociation : Bulk pKa from NMR, surface pKa from MD.
- Salt effects : Modified adsorption constants (KA⁻ = 55 M⁻¹ in 0.5 M NaCl vs. 11 M⁻¹ in water) .
Q. Methodological Considerations
- Experimental Design : Use D-optimal or Definitive Screening Designs (DSD) for multi-variable optimization (e.g., pH, ionic strength, concentration) .
- Data Contradictions : Resolve discrepancies in surface pKa values (e.g., 5.9 ± 0.6 vs. literature bulk pKa 4.97) by validating MD results with surface-specific spectroscopy .
Properties
IUPAC Name |
9,9,9-trideuteriononanoic acid | |
---|---|---|
Source | PubChem | |
URL | https://pubchem.ncbi.nlm.nih.gov | |
Description | Data deposited in or computed by PubChem | |
InChI |
InChI=1S/C9H18O2/c1-2-3-4-5-6-7-8-9(10)11/h2-8H2,1H3,(H,10,11)/i1D3 | |
Source | PubChem | |
URL | https://pubchem.ncbi.nlm.nih.gov | |
Description | Data deposited in or computed by PubChem | |
InChI Key |
FBUKVWPVBMHYJY-FIBGUPNXSA-N | |
Source | PubChem | |
URL | https://pubchem.ncbi.nlm.nih.gov | |
Description | Data deposited in or computed by PubChem | |
Canonical SMILES |
CCCCCCCCC(=O)O | |
Source | PubChem | |
URL | https://pubchem.ncbi.nlm.nih.gov | |
Description | Data deposited in or computed by PubChem | |
Isomeric SMILES |
[2H]C([2H])([2H])CCCCCCCC(=O)O | |
Source | PubChem | |
URL | https://pubchem.ncbi.nlm.nih.gov | |
Description | Data deposited in or computed by PubChem | |
Molecular Formula |
C9H18O2 | |
Source | PubChem | |
URL | https://pubchem.ncbi.nlm.nih.gov | |
Description | Data deposited in or computed by PubChem | |
Molecular Weight |
161.26 g/mol | |
Source | PubChem | |
URL | https://pubchem.ncbi.nlm.nih.gov | |
Description | Data deposited in or computed by PubChem | |
Synthesis routes and methods
Procedure details
Retrosynthesis Analysis
AI-Powered Synthesis Planning: Our tool employs the Template_relevance Pistachio, Template_relevance Bkms_metabolic, Template_relevance Pistachio_ringbreaker, Template_relevance Reaxys, Template_relevance Reaxys_biocatalysis model, leveraging a vast database of chemical reactions to predict feasible synthetic routes.
One-Step Synthesis Focus: Specifically designed for one-step synthesis, it provides concise and direct routes for your target compounds, streamlining the synthesis process.
Accurate Predictions: Utilizing the extensive PISTACHIO, BKMS_METABOLIC, PISTACHIO_RINGBREAKER, REAXYS, REAXYS_BIOCATALYSIS database, our tool offers high-accuracy predictions, reflecting the latest in chemical research and data.
Strategy Settings
Precursor scoring | Relevance Heuristic |
---|---|
Min. plausibility | 0.01 |
Model | Template_relevance |
Template Set | Pistachio/Bkms_metabolic/Pistachio_ringbreaker/Reaxys/Reaxys_biocatalysis |
Top-N result to add to graph | 6 |
Feasible Synthetic Routes
Disclaimer and Information on In-Vitro Research Products
Please be aware that all articles and product information presented on BenchChem are intended solely for informational purposes. The products available for purchase on BenchChem are specifically designed for in-vitro studies, which are conducted outside of living organisms. In-vitro studies, derived from the Latin term "in glass," involve experiments performed in controlled laboratory settings using cells or tissues. It is important to note that these products are not categorized as medicines or drugs, and they have not received approval from the FDA for the prevention, treatment, or cure of any medical condition, ailment, or disease. We must emphasize that any form of bodily introduction of these products into humans or animals is strictly prohibited by law. It is essential to adhere to these guidelines to ensure compliance with legal and ethical standards in research and experimentation.