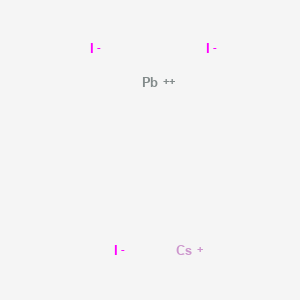
Cesium Lead Triiodide
- Click on QUICK INQUIRY to receive a quote from our team of experts.
- With the quality product at a COMPETITIVE price, you can focus more on your research.
Overview
Description
Cesium lead triiodide (CsPbI₃) is an all-inorganic halide perovskite with a cubic (α-phase) or orthorhombic (γ-phase) crystal structure at room temperature . It has gained prominence in photovoltaics due to its direct bandgap (~1.73 eV), high charge-carrier mobility, and thermal stability . Unlike organic-inorganic hybrid perovskites (e.g., methylammonium lead triiodide, MAPbI₃), CsPbI₃ lacks volatile organic cations, making it more resistant to thermal decomposition . However, its metastable α-phase tends to degrade into a non-perovskite δ-phase under ambient humidity, necessitating surface engineering or compositional tuning to stabilize the photoactive phase .
CsPbI₃-based solar cells have achieved efficiencies exceeding 20% through strategies such as thiocyanate additive engineering and surface passivation . Its optoelectronic properties, including strong light absorption and tunable bandgap, also make it suitable for light-emitting diodes (LEDs) and photodetectors .
Preparation Methods
Synthetic Routes and Reaction Conditions: Cesium lead triiodide can be synthesized using various methods, including solution-based techniques and mechanochemical synthesis. One common method involves the reaction of cesium iodide (CsI) with lead iodide (PbI₂) in a solvent such as dimethylformamide (DMF). The reaction is typically carried out at elevated temperatures to ensure complete dissolution and reaction of the precursors .
Industrial Production Methods: In industrial settings, this compound is often produced using mechanochemical synthesis. This method involves the ball milling of cesium iodide and lead iodide, which induces a solid-state reaction to form the desired perovskite phase. The mechanochemical approach is advantageous due to its simplicity, scalability, and solvent-free nature .
Chemical Reactions Analysis
Types of Reactions: Cesium lead triiodide undergoes various chemical reactions, including:
Oxidation: Exposure to oxygen can lead to the oxidation of the iodide ions, resulting in the formation of lead oxide and cesium iodide.
Reduction: Reduction reactions can occur under certain conditions, leading to the formation of lower oxidation state lead compounds.
Substitution: Halide exchange reactions can occur, where iodide ions are replaced by other halide ions such as bromide or chloride.
Common Reagents and Conditions:
Oxidation: Oxygen or air exposure at elevated temperatures.
Reduction: Reducing agents such as hydrogen gas or hydrazine.
Substitution: Halide salts such as cesium bromide or cesium chloride in a suitable solvent.
Major Products Formed:
Oxidation: Lead oxide (PbO) and cesium iodide (CsI).
Reduction: Lower oxidation state lead compounds.
Substitution: Mixed halide perovskites such as cesium lead bromide (CsPbBr₃) or cesium lead chloride (CsPbCl₃).
Scientific Research Applications
Photovoltaic Applications
1.1 Perovskite Solar Cells (PSCs)
CsPbI3 is primarily recognized for its application in perovskite solar cells, where it serves as a light-absorbing layer. The material exhibits a suitable bandgap (~1.73 eV), high absorption coefficient, and excellent charge-carrier mobility, making it ideal for solar energy conversion.
- Efficiency : Recent advancements have led to efficiencies exceeding 23% for CsPbI3-based solar cells. For instance, a study reported a conversion efficiency of 21.8%, maintaining 97% of its initial efficiency after 440 hours of continuous illumination .
- Stability : The thermal and chemical stability of CsPbI3 is superior to traditional organic-inorganic hybrid perovskites like MAPbI3, which enhances its viability in practical applications .
Property | CsPbI3 Value | Comparison with MAPbI3 |
---|---|---|
Bandgap | ~1.73 eV | ~1.55 eV |
Efficiency | >23% | ~20% |
Stability | High | Moderate |
1.2 Hybrid Systems
Hybrid systems combining CsPbI3 with other materials (e.g., formamidinium) have been explored to enhance performance further. A recent study indicated that integrating germanium into the structure improved stability and efficiency .
Photodetectors
CsPbI3 is also utilized in photodetectors due to its excellent light absorption properties and fast response times.
- Performance : Devices made from CsPbI3 have shown promising results in detecting visible light with high sensitivity and fast response times, making them suitable for applications in imaging and sensing technologies.
Light Emitting Devices
The photoluminescence properties of CsPbI3 allow it to be used in light-emitting diodes (LEDs). The tunability of its emission spectrum through compositional adjustments enables the development of LEDs with specific color outputs.
Case Studies
4.1 Efficiency Improvement through Material Engineering
A study focused on optimizing the morphology of CsPbI3 thin films demonstrated that controlling the crystallization process could significantly enhance device performance. By employing molten salts during crystallization, researchers achieved over 21% efficiency in solar cells .
4.2 Surface Ligand Engineering
Research has shown that surface ligands can stabilize different phases of CsPbI3, affecting its electronic properties and overall performance in devices . This approach allows for fine-tuning the material's characteristics for specific applications.
Mechanism of Action
The mechanism by which cesium lead triiodide exerts its effects is primarily related to its electronic structure and optoelectronic properties. The compound has a perovskite crystal structure, which allows for efficient light absorption and charge transport. The presence of cesium and lead ions in the lattice contributes to the formation of a stable and efficient charge transport network. The iodide ions play a crucial role in the compound’s light absorption properties, enabling it to capture a broad spectrum of light .
Comparison with Similar Compounds
Methylammonium Lead Triiodide (MAPbI₃)
- Structural Stability : MAPbI₃ exhibits superior phase stability under ambient conditions compared to CsPbI₃ but degrades rapidly under heat (>85°C) due to organic cation volatility . Oxygen plasma treatment of MAPbI₃ films doped with CsPbI₃ quantum dots (QDs) enhances light-harvesting efficiency at low power (20 W) but causes severe degradation at higher power (80 W) due to MAPbI₃ decomposition .
- Optoelectronic Properties : MAPbI₃ has a narrower bandgap (~1.55 eV), enabling broader visible-light absorption. However, CsPbI₃ demonstrates higher charge-carrier mobility and thermal stability .
- Device Performance : MAPbI₃ solar cells initially achieved higher efficiencies (up to 22%) but suffer from long-term instability. CsPbI₃ devices, while slightly less efficient (~21%), show improved operational stability under thermal stress .
Formamidinium Lead Triiodide (FAPbI₃)
- Phase Stability : FAPbI₃ has a near-ideal bandgap (~1.48 eV) but suffers from phase instability, transitioning into a photoinactive hexagonal δ-phase at room temperature. Mixed cation systems (e.g., FA-Cs) stabilize the perovskite phase, combining the benefits of both materials .
- Efficiency : FAPbI₃-based devices achieve efficiencies over 25%, but mixed Cs-FA systems balance efficiency (23%) with enhanced phase and thermal stability .
Tin-Based Perovskites (e.g., CsSnI₃, FASnI₃)
- Toxicity and Bandgap : Tin-based perovskites (e.g., CsSnI₃, bandgap ~1.3 eV) are lead-free but exhibit lower oxidation resistance and rapid Sn²⁺ → Sn⁴⁺ degradation .
- Device Performance: CsSn₀.₅Ge₀.₅I₃ solar cells achieve 7.11% efficiency, significantly lower than CsPbI₃ .
Mixed Cation/Anion Systems
- Cs-FA-Pb-I Systems : Mixing Cs⁺ and FA⁺ cations improves phase stability and reduces defect density. For example, α-FA-CsPbI₃ stabilized with germanium additives retains its phase under humidity and achieves 15% efficiency .
- Halide Mixing : Incorporating bromide (Br⁻) enhances moisture resistance but widens the bandgap. CsPbI₂Br, for instance, achieves 17% efficiency with improved stability .
Data Tables
Table 1: Structural and Optoelectronic Properties
*Stabilized via surface engineering or additives.
Table 2: Stability Under Environmental Stress
*Improved with hydrophobic coatings or alloying.
Key Research Findings
Phase Stabilization : Surface engineering (e.g., thiocyanate molten salts) and cation mixing (Cs/FA) mitigate CsPbI₃’s phase instability, enabling >20% efficiency .
Tin Perovskite Limitations : Despite being lead-free, tin-based perovskites suffer from Sn²⁺ oxidation and poor efficiency (<10%) .
Hybrid Systems : FA-Cs-Pb-I systems combine the narrow bandgap of FAPbI₃ with the stability of CsPbI₃, achieving 23% efficiency with minimal degradation .
Biological Activity
Cesium lead triiodide (CsPbI3) is a perovskite material that has gained significant attention in recent years, particularly in the field of optoelectronics and photovoltaics. Its unique properties, such as high absorption coefficients and tunable band gaps, make it a promising candidate for various applications, including solar cells, light-emitting devices, and photodetectors. However, understanding its biological activity is crucial for assessing its safety and potential ecological impact.
Chemical Structure and Properties
This compound has a chemical formula of CsPbI3, where cesium (Cs) acts as the A-site cation, lead (Pb) as the B-site cation, and iodine (I) as the anion. The structure can be represented as follows:
Physical Properties
- Crystal Structure : Cubic at high temperatures, orthorhombic at room temperature.
- Band Gap : Approximately 1.73 eV in its cubic form.
- Solubility : Soluble in polar solvents like dimethylformamide (DMF).
Toxicological Studies
Research indicates that this compound exhibits varying degrees of toxicity depending on exposure levels and routes. Key findings include:
- Cytotoxicity : Studies have shown that CsPbI3 can induce cytotoxic effects in certain cell lines. For instance, exposure to CsPbI3 nanoparticles resulted in decreased cell viability in human lung fibroblast cells (WI-38) at concentrations above 50 µg/mL .
- Genotoxicity : In vitro assays have indicated that this compound may cause DNA damage in human lymphocytes, raising concerns about its mutagenic potential .
Environmental Impact
The environmental implications of CsPbI3 are also a subject of ongoing research:
- Aquatic Toxicity : Preliminary studies suggest that CsPbI3 can be toxic to aquatic organisms. For example, exposure to this compound has been linked to adverse effects on fish embryos and larvae .
- Bioaccumulation Potential : The potential for bioaccumulation in food chains is another area of concern, necessitating further investigation into the long-term ecological effects of CsPbI3.
Case Study 1: Cytotoxic Effects on Human Cells
A study evaluated the cytotoxic effects of CsPbI3 on various human cell lines, including breast cancer (MCF-7) and lung fibroblast (WI-38) cells. The results showed a dose-dependent decrease in cell viability with IC50 values calculated at approximately 40 µg/mL for MCF-7 cells and 50 µg/mL for WI-38 cells.
Cell Line | IC50 (µg/mL) | % Viability at 100 µg/mL |
---|---|---|
MCF-7 | 40 | 25% |
WI-38 | 50 | 30% |
Case Study 2: Environmental Toxicity Assessment
In another study assessing the environmental impact of CsPbI3, researchers exposed zebrafish embryos to varying concentrations of the compound. The findings indicated significant developmental abnormalities at concentrations above 10 µg/mL.
Concentration (µg/mL) | % Abnormal Development |
---|---|
0 | 5% |
10 | 20% |
50 | 60% |
Q & A
Basic Research Questions
Q. What are the common crystal phases of CsPbI3, and how do they influence optoelectronic stability?
CsPbI3 exhibits three primary phases: cubic (α), tetragonal (β), and orthorhombic (γ). The γ-phase is the most stable under ambient conditions due to its non-perovskite orthorhombic structure, which minimizes degradation from moisture and thermal stress . Stability is critical for photovoltaic applications, as phase transitions (e.g., α → γ) degrade light absorption and charge transport. Researchers should monitor phase purity using X-ray diffraction (XRD) and UV-vis spectroscopy, ensuring synthesis conditions (e.g., temperature > 320°C for α-phase stabilization) align with target applications .
Q. What methodologies are recommended for synthesizing high-purity CsPbI3 thin films?
A two-step vapor-assisted solution process is widely used:
- Step 1 : Spin-coat a PbI2 precursor layer.
- Step 2 : Expose to CsI vapor at controlled humidity and temperature (e.g., 150°C) to facilitate crystallization. This method minimizes defects and enhances grain size, critical for charge mobility . Alternative approaches, such as antisolvent dripping (e.g., using chlorobenzene), can improve film uniformity but require precise solvent engineering to avoid PbI2 residues .
Q. How do surface terminations (CsI vs. PbI2) affect CsPbI3 electronic properties?
First-principles calculations reveal that CsI-terminated surfaces are more thermodynamically stable than PbI2-terminated ones. CsI surfaces exhibit surface states near the conduction band edge, enhancing electron extraction in solar cells. In contrast, PbI2 termination introduces mid-gap states that act as recombination centers. Researchers should use surface-sensitive techniques like X-ray photoelectron spectroscopy (XPS) and Kelvin probe force microscopy (KPFM) to verify termination effects .
Advanced Research Questions
Q. What strategies mitigate phase instability in CsPbI3 under operational conditions?
- Additive Engineering : Incorporating dimethylammonium (DMA<sup>+</sup>) or trimethylgermanium chloride (TGC) into the precursor solution stabilizes the α-phase by reducing lattice strain. For example, TGC addition achieves 100% initial efficiency retention after 3,000 hours under illumination .
- Strain Modulation : Epitaxial growth on lattice-matched substrates (e.g., TiO2) suppresses phase transitions via compressive strain. Synchrotron-based grazing-incidence XRD is recommended for strain analysis .
Q. How can surface reconstruction mechanisms be controlled to minimize interfacial losses in CsPbI3 solar cells?
Surface reconstructions in CsPbI3 often involve Pb-I bond breakage, creating undercoordinated Pb atoms. Passivation with thiocyanate (SCN<sup>−</sup>) ligands or post-treatment with Pb(SCN)2 reduces defect density by filling iodine vacancies. Transient absorption spectroscopy (TAS) and density functional theory (DFT) simulations are critical for mapping defect dynamics .
Q. What experimental approaches resolve contradictions in reported bandgap values for CsPbI3?
Bandgap variations (1.73–1.82 eV) arise from differences in crystallite size, strain, and surface termination. To resolve discrepancies:
- Use temperature-dependent photoluminescence (TDPL) to distinguish between intrinsic bandgap and defect-related emissions.
- Cross-validate with ellipsometry to account for thin-film interference effects .
Q. How does grain orientation in CsPbI3 films influence photovoltaic performance?
High-temperature processing (>300°C) induces preferential (111)-oriented grains in CsPbI3, which enhance charge transport along the [111] crystallographic direction. Electron backscatter diffraction (EBSD) and polarized Raman spectroscopy are essential for correlating texture with device metrics (e.g., fill factor) .
Q. Methodological Guidelines
- Data Contradiction Analysis : When conflicting results arise (e.g., stability claims), replicate experiments using identical synthesis protocols and characterize with multiple techniques (e.g., XRD for phase purity, time-resolved photoluminescence for carrier lifetime) .
- Experimental Design : For doping studies, employ combinatorial libraries with gradient compositions to efficiently map additive effects on stability and efficiency .
- Surface Characterization : Combine atomic-resolution scanning transmission electron microscopy (STEM) with surface phase diagram (SPD) analysis to predict stable surface configurations under varying chemical potentials .
Properties
CAS No. |
18041-25-3 |
---|---|
Molecular Formula |
CsI3Pb |
Molecular Weight |
721 g/mol |
IUPAC Name |
cesium;lead(2+);triiodide |
InChI |
InChI=1S/Cs.3HI.Pb/h;3*1H;/q;;;;+3/p-3 |
InChI Key |
JGJAXMBIKSSTFC-UHFFFAOYSA-K |
SMILES |
[I-].[I-].[I-].[Cs+].[Pb+2] |
Canonical SMILES |
I[Pb](I)I.[Cs] |
Origin of Product |
United States |
Disclaimer and Information on In-Vitro Research Products
Please be aware that all articles and product information presented on BenchChem are intended solely for informational purposes. The products available for purchase on BenchChem are specifically designed for in-vitro studies, which are conducted outside of living organisms. In-vitro studies, derived from the Latin term "in glass," involve experiments performed in controlled laboratory settings using cells or tissues. It is important to note that these products are not categorized as medicines or drugs, and they have not received approval from the FDA for the prevention, treatment, or cure of any medical condition, ailment, or disease. We must emphasize that any form of bodily introduction of these products into humans or animals is strictly prohibited by law. It is essential to adhere to these guidelines to ensure compliance with legal and ethical standards in research and experimentation.