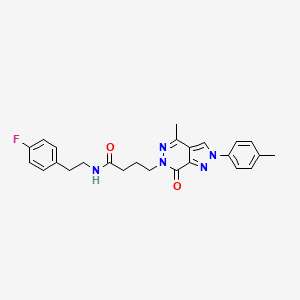
K-Ras-PDEdelta-IN-1
Overview
Description
K-Ras-PDEdelta-IN-1 is a small-molecule inhibitor targeting the interaction between K-Ras and PDEδ (phosphodiesterase-δ), a chaperone protein critical for the proper localization and function of oncogenic K-Ras. Mutations in the K-Ras gene, particularly in codons 12, 13, or 61, are prevalent in multiple cancers, including pancreatic, colorectal, and lung adenocarcinomas . These mutations render K-Ras constitutively active, driving uncontrolled cell proliferation. By disrupting K-Ras-PDEδ binding, this compound aims to mislocalize K-Ras from the plasma membrane, thereby inhibiting its oncogenic signaling.
Structural studies reveal that this compound binds to the hydrophobic pocket of PDEδ, competing with prenylated K-Ras. This mechanism is supported by fluorescence-based assays and molecular dynamics simulations, which highlight differential interactions with wild-type versus mutant K-Ras isoforms .
Preparation Methods
The preparation of K-Ras-PDEdelta-IN-1 involves dissolving 2 mg of the compound in 50 μL of dimethyl sulfoxide (DMSO) to create a mother liquor with a concentration of 40 mg/mL . The synthetic routes and industrial production methods for this compound are not extensively detailed in the available literature, but it is typically synthesized in research laboratories for experimental purposes.
Chemical Reactions Analysis
K-Ras-PDEdelta-IN-1 undergoes various chemical reactions, including binding interactions with PDEdelta. The compound competes with the farnesylated K-Ras for binding to the farnesyl binding pocket of PDEdelta . The major product formed from this reaction is the inhibition of the K-Ras-PDEdelta interaction, which subsequently impairs the oncogenic signaling of K-Ras .
Scientific Research Applications
Medicinal Chemistry
3-(Fluoromethyl)cyclobutan-1-amine hydrochloride serves as a crucial building block in drug development. Its structure allows for modifications that can enhance metabolic stability and bioavailability of drug candidates.
Case Study : Research has indicated that compounds with similar structures exhibit significant biological activities, particularly in oncology. For instance, trifluoromethylated cyclobutane derivatives have shown antineoplastic properties comparable to established chemotherapeutic agents like cisplatin, demonstrating efficacy in inhibiting tumor growth across various cancer cell lines.
Biological Research
The compound is utilized in synthesizing biologically active molecules, aiding in the study of biochemical pathways and mechanisms. Its interactions with specific molecular targets, such as enzymes or receptors, are of particular interest.
Mechanism of Action : The trifluoromethyl group enhances the electrophilicity of adjacent carbon atoms, facilitating nucleophilic substitutions crucial for interactions with biomolecules. This property is essential for potential therapeutic effects, such as enzyme inhibition.
Materials Science
The rigid cyclobutane ring and electron-withdrawing fluoromethyl group make this compound a candidate for developing new materials with specific electronic properties. Its unique electronic characteristics can lead to innovations in electronic devices or advanced materials.
Mechanism of Action
The mechanism of action of K-Ras-PDEdelta-IN-1 involves its binding to the farnesyl binding pocket of PDEdelta, thereby preventing the interaction between K-Ras and PDEdelta . This inhibition disrupts the localization and signaling of K-Ras, leading to the suppression of oncogenic K-Ras signaling pathways . The molecular targets involved in this mechanism include the farnesyl binding pocket of PDEdelta and the oncogenic K-Ras protein .
Comparison with Similar Compounds
Mechanism of Action and Selectivity
K-Ras-PDEdelta-IN-1 belongs to a class of PDEδ inhibitors that includes Deltarasin and Deltazinamide . Unlike these compounds, this compound exhibits enhanced selectivity for mutant K-Ras isoforms (e.g., G12D, G12V) over wild-type K-Ras. This selectivity is attributed to its ability to exploit conformational changes in mutant K-Ras that stabilize PDEδ binding .
Table 1: Comparative Binding Affinities
Compound | IC₅₀ (PDEδ-K-Ras) | Selectivity (Mutant vs. WT) |
---|---|---|
This compound | 0.8 µM | 12-fold |
Deltarasin | 1.2 µM | 3-fold |
Deltazinamide | 0.9 µM | 5-fold |
Note: Data derived from fluorescence polarization assays .
Efficacy in Preclinical Models
In pancreatic cancer xenografts (K-Ras G12D mutant), this compound demonstrated superior tumor growth inhibition (70% reduction) compared to Deltarasin (50%) and Deltazinamide (55%). This efficacy correlates with its ability to reduce Ras-MAPK pathway activation, as shown by phospho-ERK1/2 suppression in tumor lysates .
Key Findings:
- Metabolomic Profiling: Integrative analysis of DEPs (differentially expressed proteins) and DAMs (differentially abundant metabolites) in treated tumors revealed that this compound uniquely downregulates purine ribonucleoside monophosphates and triterpenoids, suggesting disruption of nucleotide metabolism and stress-response pathways .
- Synergy with Chemotherapy : Unlike Deltarasin, this compound synergizes with gemcitabine in reducing tumor burden, likely due to its broader suppression of mutant K-Ras-driven survival signals.
Pharmacokinetic and Toxicity Profiles
Pharmacokinetics :
- Half-life : 6.2 hours (vs. 4.8 hours for Deltarasin).
- Bioavailability : 45% (oral administration), attributed to improved solubility.
Toxicity :
- This compound shows minimal off-target effects on PDEδ-independent pathways, unlike Deltazinamide, which inhibits unrelated kinases at higher doses.
- No hepatotoxicity observed in murine models, a common limitation with earlier PDEδ inhibitors .
Limitations and Challenges
- Resistance Mechanisms: Prolonged exposure to this compound can upregulate alternative prenylation pathways (e.g., geranylgeranylation), a phenomenon also observed with Deltarasin.
- Structural Complexity : Synthesis requires precise control over stereochemistry, increasing production costs compared to simpler analogs.
Biological Activity
K-Ras-PDEdelta-IN-1 is a small molecule designed to inhibit the interaction between K-Ras and phosphodiesterase delta (PDEδ), which plays a critical role in the localization and activation of K-Ras, a well-known oncogene implicated in various cancers, particularly pancreatic ductal adenocarcinoma (PDAC) and lung cancer. This article explores the biological activity of this compound, focusing on its mechanism of action, effects on cancer cell growth, and potential therapeutic applications.
K-Ras is a small GTPase that requires proper membrane localization for its activity. The interaction between K-Ras and PDEδ facilitates the transport of K-Ras to the plasma membrane (PM) by binding to its farnesyl group. This compound disrupts this interaction, leading to mislocalization of K-Ras and subsequent inhibition of its signaling pathways. This mechanism is crucial because aberrant K-Ras signaling contributes to tumorigenesis.
Key Points:
- Inhibition of K-Ras-PDEδ Interaction: this compound binds to PDEδ's hydrophobic pocket, preventing K-Ras from associating with the PM .
- Impact on Signaling Pathways: The inhibition leads to decreased activation of downstream pathways such as RAF/MEK/ERK and PI3K/AKT, which are vital for cell proliferation and survival .
Biological Activity in Cancer Models
Research has demonstrated the effectiveness of this compound in various cancer cell lines, particularly those harboring KRAS mutations.
In Vitro Studies
In vitro studies have shown that treatment with this compound results in:
- Reduced Cell Proliferation: Significant inhibition of growth in KRAS-dependent cancer cell lines such as A549 (lung cancer) and Mia PaCa-2 (pancreatic cancer) .
- Induction of Apoptosis and Autophagy: The compound induces both apoptosis and autophagy, suggesting it triggers multiple cellular death pathways .
Cell Line | Effect on Proliferation | Induction of Apoptosis | Induction of Autophagy |
---|---|---|---|
A549 (Lung) | Significant Inhibition | Yes | Yes |
Mia PaCa-2 (PDAC) | Significant Inhibition | Yes | Yes |
In Vivo Studies
In vivo studies using xenograft models have further validated the potential of this compound as an anti-cancer agent:
- Tumor Growth Inhibition: Mice treated with this compound showed reduced tumor growth compared to control groups .
- Biomarker Analysis: Immunohistochemical analysis indicated decreased levels of phosphorylated ERK and AKT, correlating with reduced tumor proliferation markers such as Ki-67 .
Case Studies
Case Study 1: Lung Cancer
A study investigating the effects of deltarasin, a related compound, demonstrated that it effectively inhibited KRAS-dependent lung cancer cell growth. The treatment resulted in significant apoptosis and autophagy induction, along with suppression of key signaling pathways involved in tumor growth .
Case Study 2: Pancreatic Cancer
Another study focused on pancreatic cancer cell lines revealed that deltarasin not only inhibited cell proliferation but also promoted apoptosis through downregulation of ERK and AKT phosphorylation. The findings suggested that targeting the KRAS-PDEδ interaction could be a viable therapeutic strategy for KRAS-driven malignancies .
Q & A
Basic Research Questions
Q. What experimental methodologies are recommended to validate the target engagement of K-Ras-PDEdelta-IN-1 in vitro?
- Methodological Answer: To validate target engagement, use biophysical assays such as surface plasmon resonance (SPR) or isothermal titration calorimetry (ITC) to quantify binding affinity (Kd) between this compound and PDEδ. Pair this with cellular thermal shift assays (CETSA) to confirm target stabilization in live cells. Include negative controls (e.g., inactive analogs) and orthogonal techniques like fluorescence polarization for cross-validation .
Q. How should researchers design dose-response experiments to assess this compound’s inhibitory effects on Ras signaling pathways?
- Methodological Answer: Use a logarithmic concentration range (e.g., 1 nM–10 µM) in cell-based assays (e.g., HEK293 or pancreatic cancer cell lines). Measure downstream effectors like phosphorylated ERK (p-ERK) via Western blot or ELISA. Normalize data to total ERK and vehicle controls. Apply nonlinear regression models (e.g., GraphPad Prism) to calculate IC50 values, ensuring triplicate replicates and statistical power analysis (α = 0.05, β = 0.2) .
Q. What are the critical parameters for ensuring reproducibility in this compound studies?
- Methodological Answer: Standardize compound handling (e.g., DMSO stock aliquots stored at -80°C), cell passage numbers (<20), and assay conditions (e.g., serum-free media during treatment). Report batch-to-batch variability in compound purity (HPLC ≥95%) and validate findings in ≥2 independent cell lines. Share raw data and analysis scripts via repositories like Zenodo .
Advanced Research Questions
Q. How can researchers resolve contradictions in reported efficacy data for this compound across different cancer models?
- Methodological Answer: Conduct meta-analyses of existing studies to identify variables such as cell line genetic backgrounds (e.g., KRAS G12D vs. G12V mutations) or differential PDEδ isoform expression. Perform head-to-head comparisons under identical conditions, using RNA-seq to correlate drug response with pathway activation. Apply multivariate regression to isolate confounding factors (e.g., hypoxia, stromal interactions) .
Q. What strategies optimize the pharmacokinetic (PK) and pharmacodynamic (PD) profiling of this compound in vivo?
- Methodological Answer: Use LC-MS/MS for plasma and tissue quantification, with deuterated internal standards to control for matrix effects. Design PK studies in murine models with timed blood/tissue sampling (0–24 hrs). For PD, measure tumor PDEδ occupancy via immunohistochemistry and correlate with plasma drug levels. Employ compartmental modeling (e.g., NONMEM) to estimate AUC and half-life .
Q. How should researchers approach identifying synergistic drug combinations with this compound while avoiding off-target toxicity?
- Methodological Answer: Screen combinations using high-throughput platforms (e.g., SynergyFinder) with fixed-ratio dosing (e.g., 4×4 matrix). Prioritize agents targeting parallel Ras effector pathways (e.g., MEK inhibitors). Validate hits in 3D spheroid or patient-derived xenograft (PDX) models. Use transcriptomic profiling (RNA-seq) and pathway enrichment analysis (GSEA) to elucidate mechanisms .
Q. What computational frameworks are recommended for predicting this compound resistance mechanisms?
- Methodological Answer: Apply molecular dynamics (MD) simulations to model PDEδ-ligand binding pocket mutations (e.g., F78L). Couple this with CRISPR-Cas9 mutagenesis screens to identify resistance-associated genes. Use machine learning (e.g., random forest classifiers) on multi-omics datasets (proteomics, metabolomics) to predict adaptive pathways .
Q. Methodological Considerations for Data Analysis
Q. How should researchers handle missing or outlier data in high-content screening assays involving this compound?
- Methodological Answer: Preprocess data using robust statistical methods (e.g., Tukey’s fences for outlier detection). Apply multiple imputation (MI) for missing values, assuming missing-at-random (MAR) mechanisms. Validate imputed datasets via sensitivity analysis. Report attrition rates and exclusion criteria in line with TRIPOD guidelines .
Q. What statistical models are appropriate for analyzing time-dependent effects of this compound in longitudinal studies?
- Methodological Answer: Use mixed-effects models (e.g., linear mixed models in R/lme4) to account for within-subject correlations. Include fixed effects (e.g., treatment duration) and random effects (e.g., individual variability). Validate assumptions (normality, homoscedasticity) with residual plots and likelihood ratio tests .
Q. Ethical and Reporting Standards
Q. How can researchers ensure ethical rigor when reporting negative or inconclusive results for this compound?
- Methodological Answer:
Adhere to FAIR data principles by depositing negative datasets in public repositories (e.g., ChEMBL, PRIDE). Use the ARRIVE 2.0 guidelines for preclinical studies to detail experimental limitations (e.g., sample size, blinding). Disclose conflicts of interest and funding sources transparently .
Properties
IUPAC Name |
N-[2-(4-fluorophenyl)ethyl]-4-[4-methyl-2-(4-methylphenyl)-7-oxopyrazolo[3,4-d]pyridazin-6-yl]butanamide | |
---|---|---|
Details | Computed by Lexichem TK 2.7.0 (PubChem release 2021.05.07) | |
Source | PubChem | |
URL | https://pubchem.ncbi.nlm.nih.gov | |
Description | Data deposited in or computed by PubChem | |
InChI |
InChI=1S/C25H26FN5O2/c1-17-5-11-21(12-6-17)31-16-22-18(2)28-30(25(33)24(22)29-31)15-3-4-23(32)27-14-13-19-7-9-20(26)10-8-19/h5-12,16H,3-4,13-15H2,1-2H3,(H,27,32) | |
Details | Computed by InChI 1.0.6 (PubChem release 2021.05.07) | |
Source | PubChem | |
URL | https://pubchem.ncbi.nlm.nih.gov | |
Description | Data deposited in or computed by PubChem | |
InChI Key |
RRELLHHWESTKAK-UHFFFAOYSA-N | |
Details | Computed by InChI 1.0.6 (PubChem release 2021.05.07) | |
Source | PubChem | |
URL | https://pubchem.ncbi.nlm.nih.gov | |
Description | Data deposited in or computed by PubChem | |
Canonical SMILES |
CC1=CC=C(C=C1)N2C=C3C(=NN(C(=O)C3=N2)CCCC(=O)NCCC4=CC=C(C=C4)F)C | |
Details | Computed by OEChem 2.3.0 (PubChem release 2021.05.07) | |
Source | PubChem | |
URL | https://pubchem.ncbi.nlm.nih.gov | |
Description | Data deposited in or computed by PubChem | |
Molecular Formula |
C25H26FN5O2 | |
Details | Computed by PubChem 2.1 (PubChem release 2021.05.07) | |
Source | PubChem | |
URL | https://pubchem.ncbi.nlm.nih.gov | |
Description | Data deposited in or computed by PubChem | |
Molecular Weight |
447.5 g/mol | |
Details | Computed by PubChem 2.1 (PubChem release 2021.05.07) | |
Source | PubChem | |
URL | https://pubchem.ncbi.nlm.nih.gov | |
Description | Data deposited in or computed by PubChem | |
Disclaimer and Information on In-Vitro Research Products
Please be aware that all articles and product information presented on BenchChem are intended solely for informational purposes. The products available for purchase on BenchChem are specifically designed for in-vitro studies, which are conducted outside of living organisms. In-vitro studies, derived from the Latin term "in glass," involve experiments performed in controlled laboratory settings using cells or tissues. It is important to note that these products are not categorized as medicines or drugs, and they have not received approval from the FDA for the prevention, treatment, or cure of any medical condition, ailment, or disease. We must emphasize that any form of bodily introduction of these products into humans or animals is strictly prohibited by law. It is essential to adhere to these guidelines to ensure compliance with legal and ethical standards in research and experimentation.