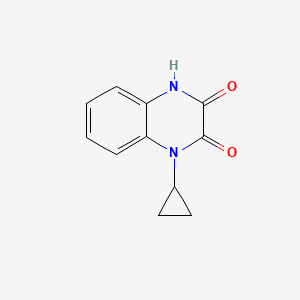
1-Cyclopropyl-1,4-dihydroquinoxaline-2,3-dione
Overview
Description
1-Cyclopropyl-1,4-dihydroquinoxaline-2,3-dione (hereafter referred to as the target compound) is a quinoxaline-2,3-dione derivative featuring a cyclopropyl substituent at the N1 position. It is synthesized via a reaction involving 1-cyclopropyl-4-hydroxy-1,4-dihydroquinoxaline-2,3-dione with triphenylphosphine in DMF at 135 °C for 6 hours, followed by purification with dichloromethane . Key spectroscopic data include a distinctive ¹H NMR signal at δ 11.92 (s, 1H) for the NH group and cyclopropyl proton signals between δ 0.66–1.30 . This compound serves as an intermediate in synthesizing tetrahydroquinoxaline derivatives, such as 1-cyclopropyl-1,2,3,4-tetrahydroquinoxaline (compound 12), via borane-THF reduction .
Preparation Methods
Synthetic Routes and Reaction Conditions
1-Cyclopropyl-1,4-dihydroquinoxaline-2,3-dione can be synthesized through a one-pot reaction involving substituted o-phenylenediamine and oxalic acid under solvent-free conditions . This method is efficient and environmentally friendly, utilizing simple grinding techniques at room temperature .
Industrial Production Methods
While specific industrial production methods for this compound are not widely documented, the principles of green chemistry and atom economy are often applied to scale up laboratory synthesis methods for industrial use .
Chemical Reactions Analysis
Types of Reactions
1-Cyclopropyl-1,4-dihydroquinoxaline-2,3-dione undergoes various chemical reactions, including:
Oxidation: This reaction can lead to the formation of quinoxaline derivatives.
Reduction: This reaction can produce dihydroquinoxaline derivatives.
Substitution: This reaction can introduce different functional groups into the quinoxaline ring.
Common Reagents and Conditions
Oxidation: Common oxidizing agents include potassium permanganate and hydrogen peroxide.
Reduction: Common reducing agents include sodium borohydride and lithium aluminum hydride.
Substitution: Common reagents include halogens and alkylating agents.
Major Products Formed
The major products formed from these reactions include various quinoxaline derivatives, which have applications in pharmaceuticals and materials science .
Scientific Research Applications
1-Cyclopropyl-1,4-dihydroquinoxaline-2,3-dione has several scientific research applications:
Chemistry: Used as a building block for synthesizing complex organic molecules.
Biology: Studied for its potential as an enzyme inhibitor.
Medicine: Investigated for its potential therapeutic effects, particularly in neurological disorders.
Industry: Used in the development of new materials with unique properties.
Mechanism of Action
The mechanism of action of 1-Cyclopropyl-1,4-dihydroquinoxaline-2,3-dione involves its interaction with specific molecular targets, such as enzymes and receptors. It can act as an antagonist to certain receptors, thereby modulating biological pathways . The exact molecular targets and pathways involved depend on the specific application and context of use .
Comparison with Similar Compounds
Comparison with Similar Quinoxaline-2,3-dione Derivatives
Table 1: Key Structural and Functional Comparisons
Crystallographic and Supramolecular Features
- Crystal Packing: Derivatives like 1,4-dihexyl-1,4-dihydroquinoxaline-2,3-dione (FECROX) exhibit layered packing stabilized by van der Waals interactions due to long alkyl chains . The target compound’s cyclopropyl group likely induces tighter packing, as seen in similar structures .
- Hydrogen Bonding: Quinoxaline-2,3-diones generally form hydrogen bonds via carbonyl groups (e.g., ANQX with GluR2 S1S2J domain ). The target compound’s NH group (δ 11.92) may participate in similar interactions during receptor binding .
Biological Activity
1-Cyclopropyl-1,4-dihydroquinoxaline-2,3-dione (CPQ) is a heterocyclic compound that has garnered significant attention in medicinal chemistry due to its diverse biological activities. The molecular formula of CPQ is CHNO, and it features a cyclopropyl group attached to a quinoxaline core. This unique structure contributes to its reactivity and potential therapeutic applications, particularly in the fields of oncology and neurology.
Chemical Structure and Properties
The structural characteristics of CPQ play a crucial role in its biological activity. The presence of two nitrogen atoms in the quinoxaline ring and carbonyl groups enhances its interaction with biological targets.
Compound | Molecular Formula | Structural Features | Notable Activities |
---|---|---|---|
This compound | CHNO | Cyclopropyl group, quinoxaline core | Anticancer, antimicrobial, MAO-B inhibition |
Antimicrobial Activity
Research indicates that CPQ exhibits significant antimicrobial properties. It has been evaluated against various bacterial strains and has shown promising results in inhibiting growth. For instance, studies have demonstrated its effectiveness against both gram-positive and gram-negative bacteria, suggesting potential applications in treating infections.
Anticancer Properties
CPQ has also been investigated for its anticancer effects. Preliminary studies reveal that it possesses cytotoxic activity against several cancer cell lines. The mechanism underlying this activity may involve the modulation of key signaling pathways associated with cell proliferation and apoptosis. For example, it has been shown to induce apoptosis in breast cancer cells, highlighting its potential as a therapeutic agent in oncology.
Neuropharmacological Effects
One of the most intriguing aspects of CPQ is its interaction with monoamine oxidase B (MAO-B), an enzyme involved in the metabolism of neurotransmitters. By inhibiting MAO-B, CPQ may help regulate neurotransmitter levels, which could be beneficial for treating neurodegenerative diseases such as Parkinson's disease. This property positions CPQ as a candidate for further investigation in neuropharmacology.
Study on Anticancer Activity
In a study published in 2023, researchers synthesized various derivatives of CPQ and evaluated their cytotoxic effects on human cancer cell lines. The findings indicated that certain derivatives exhibited enhanced potency compared to CPQ itself, suggesting that structural modifications can improve therapeutic efficacy.
Study on Neuropharmacological Effects
A recent investigation focused on the neuroprotective effects of CPQ in animal models of neurodegeneration. Results showed that treatment with CPQ led to significant improvements in behavioral assessments and reduced neuronal loss, supporting its potential as a neuroprotective agent.
The biological activity of CPQ is primarily attributed to its ability to interact with specific molecular targets:
- Inhibition of Enzymes : CPQ acts as an inhibitor of MAO-B, which may contribute to increased levels of neurotransmitters like dopamine.
- Induction of Apoptosis : In cancer cells, CPQ triggers apoptotic pathways through the activation of caspases and modulation of Bcl-2 family proteins.
- Antimicrobial Mechanism : The exact mechanism by which CPQ exerts antimicrobial effects is still under investigation but may involve disruption of bacterial cell wall synthesis or interference with metabolic pathways.
Summary Table of Biological Activities
Activity Type | Target/Mechanism | Findings |
---|---|---|
Antimicrobial | Various bacterial strains | Significant inhibition observed |
Anticancer | Cancer cell lines | Induces apoptosis; cytotoxic effects |
Neuropharmacological | MAO-B inhibition | Potential for treating neurodegenerative diseases |
Q & A
Q. Basic: What are the key synthetic pathways for synthesizing 1-cyclopropyl-1,4-dihydroquinoxaline-2,3-dione?
The synthesis typically involves cyclopropane functionalization and quinoxaline ring formation. A common approach is the condensation of cyclopropylamine derivatives with substituted o-phenylenediamines, followed by oxidation to form the dione moiety. For example, cyclopropane-containing precursors can react with nitro-substituted dihydroquinoxalines under reductive conditions to introduce the cyclopropyl group . Solvent choice (e.g., DMF or THF) and temperature control (60–80°C) are critical to avoid side reactions like over-oxidation or ring-opening. Yield optimization often requires iterative adjustments to stoichiometry and reaction time .
Q. Advanced: How can computational chemistry improve the design of derivatives with targeted biological activity?
Quantum mechanical calculations (e.g., DFT) can predict electronic properties (HOMO-LUMO gaps) and reactive sites, guiding substituent placement for enhanced binding affinity. For instance, introducing electron-withdrawing groups (e.g., nitro or fluoro) at specific positions may stabilize charge-transfer interactions with biological targets . Molecular docking simulations using crystal structure data (e.g., PDB ID: Y4Y) can validate hypothesized binding modes . High-throughput virtual screening of substituent libraries (e.g., cyclopropyl vs. methyl groups) further refines selectivity .
Q. Structural Analysis: What crystallographic techniques validate the molecular conformation of this compound?
Single-crystal X-ray diffraction (SC-XRD) is the gold standard. Key parameters include:
- Unit cell dimensions : Monoclinic systems (e.g., space group P2₁/n) with lattice parameters a = 7.13 Å, b = 8.42 Å, c = 15.29 Å, and β = 99.79° .
- Hydrogen bonding : Intermolecular C–H···O interactions (D···A distances: 3.25–3.40 Å) stabilize crystal packing .
- Torsion angles : Cyclopropyl ring puckering (e.g., 10–15° deviations from planarity) impacts solubility . Refinement protocols (e.g., SHELXL) ensure accuracy, with R factors < 0.1 for high-quality datasets .
Q. Data Contradictions: How can researchers resolve discrepancies in reported biological activity across studies?
Contradictions often arise from:
- Substituent effects : Minor structural variations (e.g., nitro vs. amino groups) drastically alter bioactivity. Cross-referencing synthesis protocols (e.g., purity ≥98% by HPLC ) and assay conditions (e.g., cell line specificity) is essential .
- Experimental design : Statistical methods like Design of Experiments (DoE) can isolate critical variables (e.g., pH, temperature) and reduce noise . For example, a 2³ factorial design might reveal solvent polarity as a key factor in cytotoxicity assays .
Q. Reactivity: What solvent systems optimize its reactivity in nucleophilic substitution reactions?
Polar aprotic solvents (e.g., DMSO, acetonitrile) enhance nucleophilicity due to high dielectric constants. However, cyclopropane strain increases susceptibility to ring-opening in protic solvents (e.g., water/methanol mixtures). Kinetic studies show reaction rates in DMF are 3× faster than in THF due to better stabilization of transition states . Solubility can be improved via co-solvents (e.g., 10% ethanol in water), as evidenced by Hirshfeld surface analysis of crystal packing .
Q. Advanced Design: How can derivatives be engineered for improved photostability or redox properties?
- Photostability : Introducing electron-donating groups (e.g., methoxy) at the 6- or 7-position reduces π→π* excitation, minimizing photodegradation. UV-Vis spectra (λmax ~300 nm) and TD-DFT calculations guide modifications .
- Redox tuning : Cyclic voltammetry reveals reversible oxidation peaks at ~0.8 V (vs. Ag/AgCl). Substituents like fluorine lower the LUMO energy, enhancing electron-accepting capacity for catalytic applications .
Q. Methodological Gaps: What unresolved challenges exist in characterizing its reaction intermediates?
- Transient species : Short-lived intermediates (e.g., radical anions) require advanced techniques like time-resolved FTIR or EPR spectroscopy.
- Stereochemical control : Chiral cyclopropane derivatives often racemize during synthesis. Enantioselective routes using chiral auxiliaries (e.g., Evans oxazolidinones) are under exploration .
Q. Analytical Validation: How to ensure purity and structural fidelity in batch synthesis?
- Chromatography : Reverse-phase HPLC (C18 column, 70:30 acetonitrile/water) with UV detection at 254 nm resolves impurities (<1% area) .
- Mass spectrometry : High-resolution ESI-MS (e.g., [M+H]<sup>+</sup> m/z 263.30) confirms molecular weight .
- NMR : <sup>1</sup>H NMR (DMSO-d₆) should show characteristic cyclopropyl protons as a multiplet at δ 1.2–1.5 ppm and quinoxaline NH signals at δ 10.8 ppm .
Q. Safety and Handling: What precautions are necessary for lab-scale handling?
While specific safety data are limited, analogs (e.g., 2,3-diphenylphthalazine-1,4-dione) recommend:
- PPE : Nitrile gloves, lab coats, and fume hoods for dust control .
- Storage : Under nitrogen at –20°C to prevent oxidation .
Q. Future Directions: What emerging methodologies could revolutionize its application in materials science?
- Supramolecular assembly : Hydrogen-bonded frameworks (HOFs) using 5-nitroisophthalic acid co-crystals show promise for porous materials .
- Computational-driven discovery : Machine learning models trained on reaction databases (e.g., ICSynth) predict novel cyclopropane-quinoxaline hybrids with tunable bandgaps for optoelectronics .
Properties
IUPAC Name |
4-cyclopropyl-1H-quinoxaline-2,3-dione | |
---|---|---|
Details | Computed by Lexichem TK 2.7.0 (PubChem release 2021.05.07) | |
Source | PubChem | |
URL | https://pubchem.ncbi.nlm.nih.gov | |
Description | Data deposited in or computed by PubChem | |
InChI |
InChI=1S/C11H10N2O2/c14-10-11(15)13(7-5-6-7)9-4-2-1-3-8(9)12-10/h1-4,7H,5-6H2,(H,12,14) | |
Details | Computed by InChI 1.0.6 (PubChem release 2021.05.07) | |
Source | PubChem | |
URL | https://pubchem.ncbi.nlm.nih.gov | |
Description | Data deposited in or computed by PubChem | |
InChI Key |
ONVGJIKVRHRZHI-UHFFFAOYSA-N | |
Details | Computed by InChI 1.0.6 (PubChem release 2021.05.07) | |
Source | PubChem | |
URL | https://pubchem.ncbi.nlm.nih.gov | |
Description | Data deposited in or computed by PubChem | |
Canonical SMILES |
C1CC1N2C3=CC=CC=C3NC(=O)C2=O | |
Details | Computed by OEChem 2.3.0 (PubChem release 2021.05.07) | |
Source | PubChem | |
URL | https://pubchem.ncbi.nlm.nih.gov | |
Description | Data deposited in or computed by PubChem | |
Molecular Formula |
C11H10N2O2 | |
Details | Computed by PubChem 2.1 (PubChem release 2021.05.07) | |
Source | PubChem | |
URL | https://pubchem.ncbi.nlm.nih.gov | |
Description | Data deposited in or computed by PubChem | |
Molecular Weight |
202.21 g/mol | |
Details | Computed by PubChem 2.1 (PubChem release 2021.05.07) | |
Source | PubChem | |
URL | https://pubchem.ncbi.nlm.nih.gov | |
Description | Data deposited in or computed by PubChem | |
Synthesis routes and methods I
Procedure details
Synthesis routes and methods II
Procedure details
Disclaimer and Information on In-Vitro Research Products
Please be aware that all articles and product information presented on BenchChem are intended solely for informational purposes. The products available for purchase on BenchChem are specifically designed for in-vitro studies, which are conducted outside of living organisms. In-vitro studies, derived from the Latin term "in glass," involve experiments performed in controlled laboratory settings using cells or tissues. It is important to note that these products are not categorized as medicines or drugs, and they have not received approval from the FDA for the prevention, treatment, or cure of any medical condition, ailment, or disease. We must emphasize that any form of bodily introduction of these products into humans or animals is strictly prohibited by law. It is essential to adhere to these guidelines to ensure compliance with legal and ethical standards in research and experimentation.