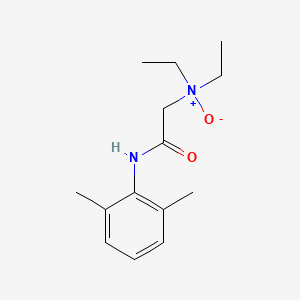
Lidocaine N-oxide
Overview
Description
Lidocaine N-oxide (chemical name: 2-(Diethyloxidoamino)-N-(2,6-dimethylphenyl)acetamide) is an oxidative metabolite of the local anesthetic lidocaine. It is formed through enzymatic or electrochemical oxidation of the tertiary amine group in lidocaine, resulting in the addition of an oxygen atom to the nitrogen . Structurally, it retains the core acetamide and aromatic moieties of lidocaine but features an N-oxide functional group, which increases its polarity compared to the parent compound . This compound is identified as a pharmacologically relevant metabolite, though its direct anesthetic activity is less pronounced than lidocaine itself .
Preparation Methods
Electrochemical Oxidation Under Basic Conditions
Electrochemical oxidation has emerged as a prominent method for synthesizing lidocaine N-oxide due to its mild reaction conditions and avoidance of hazardous oxidizing agents. The process involves the use of a platinum electrode in aqueous basic media, typically at pH 12, to facilitate the oxidation of the tertiary amine group in lidocaine.
Reaction Setup and Parameters
Key experimental conditions for this method include:
- Electrolyte composition : Acetonitrile (ACN) mixed with ammonium hydroxide (NH₄OH) in a 95:5 ratio.
- Electrode material : Platinum (Pt) working and counter electrodes.
- Applied potential : 1.5 V vs. Ag/AgCl reference electrode.
- Reaction duration : 30–90 minutes.
Under these conditions, lidocaine undergoes selective N-oxidation, yielding this compound alongside minor N-dealkylation products. The reaction selectivity is highly dependent on pH; at pH 12, N-oxidation dominates, whereas acidic conditions (pH < 0.5) favor aromatic hydroxylation.
Product Analysis and Yield
Liquid chromatography-tandem mass spectrometry (LC-MS/MS) is employed to characterize the products. The N-oxide derivative is identified via its mass-to-charge ratio (m/z 251/86) and retention time. Relative yields are quantified using normalized peak areas, with this compound constituting approximately 40–60% of the total oxidation products under optimal conditions.
Table 1: Effect of pH on Lidocaine Oxidation Products
pH | Dominant Product | Secondary Product | Yield (%) |
---|---|---|---|
0.5 | 3-Hydroxylidocaine | 4-Hydroxylidocaine | 80–90 |
12 | This compound | N-Deethylated lidocaine | 40–60 |
Mechanistic Pathways in N-Oxide Formation
The electrochemical N-oxidation of lidocaine is proposed to occur via two interrelated pathways involving hydroxyl radicals (·OH) and platinum-oxo species:
Hydroxyl Radical-Mediated Oxidation
Water oxidation at the platinum electrode generates adsorbed hydroxyl radicals (Eq. 1), which abstract a hydrogen atom from the tertiary amine group of lidocaine, forming a nitrogen-centered radical. Subsequent reaction with hydroxide ions (OH⁻) yields the N-oxide (Eq. 2):
$$
\text{Pt} + \text{H}2\text{O} \rightarrow \text{Pt}(\cdot\text{OH}) + \text{H}^+ + e^- \quad (1)
$$
$$
\text{Lidocaine} + \cdot\text{OH} \rightarrow \text{this compound} + \text{H}2\text{O} \quad (2)
$$
Role of Platinum-Oxo Layers
At potentials above 1.0 V, platinum electrodes develop oxide layers (PtOₓ), which act as mediators for oxygen transfer. Cyclic voltammetry studies confirm that these layers are reversibly formed and removed during the reaction (Figure 1). The oxide layers may stabilize reactive intermediates, enhancing N-oxide selectivity.
Optimization Strategies for Improved Selectivity
pH Modulation
As demonstrated in Table 1, basic conditions (pH 12) suppress competing aromatic hydroxylation and benzylic oxidation reactions. The hydroxide ion concentration directly influences the availability of OH⁻ for N-oxide formation.
Solvent Composition
A mixed solvent system of ACN/NH₄OH (95:5) balances lidocaine solubility and electrochemical stability. Reducing water content below 5% minimizes hydroxyl radical generation, thereby curtailing side reactions.
Oxygen Source Investigation
Isotopic labeling experiments using ¹⁸O-enriched water reveal that approximately 86% of the oxygen in hydroxylation products originates from water. However, dissolved molecular oxygen also contributes marginally to N-oxide formation, particularly in water-free systems.
Comparative Analysis with Alternative Methods
While electrochemical synthesis is the most extensively documented method, other approaches include:
Chemical Oxidation with Peracids
Meta-chloroperbenzoic acid (mCPBA) and hydrogen peroxide (H₂O₂) have been explored for N-oxide synthesis. However, these methods often require stoichiometric oxidants and generate acidic byproducts, complicating purification.
Enzymatic Oxidation
Cytochrome P450 enzymes catalyze the N-oxidation of lidocaine in vivo. Though environmentally benign, enzymatic methods face scalability challenges and high costs in industrial settings.
Chemical Reactions Analysis
Types of Reactions: OCT2013 undergoes a reduction reaction in the ischaemic myocardium, converting to lidocaine. This reaction is crucial for its function as an antiarrhythmic agent .
Common Reagents and Conditions: The reduction of OCT2013 to lidocaine occurs in the presence of reduced oxygen levels, typical of ischaemic conditions. This selective activation ensures that the compound remains inactive in normoxic tissues, thereby minimizing side effects .
Major Products Formed: The primary product formed from the reduction of OCT2013 is lidocaine, which then exerts its antiarrhythmic effects by blocking sodium channels in the heart .
Scientific Research Applications
OCT2013 has significant potential in the field of cardiovascular medicine. Its ability to prevent ventricular fibrillation without the systemic side effects of lidocaine makes it a promising candidate for further research and development. Current studies focus on its efficacy in animal models, with plans to extend research to human trials .
Mechanism of Action
OCT2013 acts as an ischaemia-activated prodrug. In the ischaemic myocardium, the compound is reduced to lidocaine, which then blocks sodium channels, preventing the propagation of abnormal electrical impulses that can lead to ventricular fibrillation. This selective activation ensures that the compound exerts its effects only in the affected areas, minimizing systemic side effects .
Comparison with Similar Compounds
Key Properties of Lidocaine N-Oxide
N-oxides are common metabolites or synthetic derivatives of amine-containing pharmaceuticals. Below, this compound is compared to other N-oxides in terms of pharmacokinetics, structural features, and biological roles.
Pharmacokinetic Behavior
Sorafenib N-Oxide :
- Unlike this compound, sorafenib N-oxide retains partial pharmacological activity as a metabolite of the anticancer drug sorafenib.
- In rats, sorafenib N-oxide exhibited higher plasma AUC (3.74 µg·h/mL vs. 3.05 µg·h/mL) and brain Cmax (0.19 µg/mL vs. 0.16 µg/mL) compared to the parent drug under specific conditions .
- Brain-to-plasma uptake (Kp) was similar for both sorafenib and its N-oxide, suggesting comparable blood-brain barrier penetration .
- Ranitidine N-Oxide: A known impurity in ranitidine formulations, ranitidine N-oxide is structurally distinct due to its furan and nitroethenyl groups .
Research Findings and Implications
- Electrochemical Synthesis : this compound can be generated in vitro using reactive oxygen species (ROS), replicating metabolic pathways without ethical constraints . This method parallels sorafenib N-oxide’s hepatic formation .
- Environmental Relevance : this compound (LID251) is a primary ozonation product in water treatment, with further degradation yielding carbonyl-containing derivatives (e.g., LID265) .
Biological Activity
Lidocaine N-oxide (LNO) is a metabolite of lidocaine, a widely used local anesthetic. This compound has garnered attention for its unique biological activities, pharmacokinetics, and potential therapeutic applications. This article explores the biological activity of this compound, focusing on its pharmacokinetics, metabolic pathways, and clinical implications.
Pharmacokinetics
This compound exhibits distinct pharmacokinetic properties compared to its parent compound, lidocaine. A study demonstrated that intratracheal administration of LNO resulted in plasma concentrations that were significantly higher than those of lidocaine when administered via the same route. The area under the curve (AUC) for LNO was approximately 3.3-fold greater than that of lidocaine, indicating a more prolonged systemic presence after administration .
Key Pharmacokinetic Parameters:
Parameter | Lidocaine | This compound |
---|---|---|
Clearance (mL/min/kg) | 93.0 | Not specified |
Half-life (minutes) | 28.1 | Not specified |
AUC (plasma concentration) | Baseline | 3.3-fold greater |
Systemic Bioavailability | 30% | 36.5% |
The conversion of LNO to lidocaine in lung tissues was also noted, suggesting that LNO may serve as a prodrug, enhancing the availability of lidocaine in systemic circulation .
Metabolic Pathways
Lidocaine undergoes extensive metabolism primarily in the liver, where it is converted into several metabolites, including LNO. The metabolism of lidocaine has been shown to produce potentially harmful metabolites such as 2,6-dimethyl aniline (DMA). However, studies indicate that LNO administration significantly reduces the levels of these toxic metabolites compared to direct lidocaine administration . This reduction in harmful metabolites positions LNO as a safer alternative in certain clinical scenarios.
Antiarrhythmic Properties
This compound has been investigated for its potential use as a prophylactic treatment against ventricular fibrillation (VF). Research suggests that LNO can be administered without the cardiotoxic effects commonly associated with traditional antiarrhythmics like lidocaine itself. In animal models, LNO demonstrated efficacy in preventing VF without significant adverse effects on cardiac function .
Pain Management
The combination of nitrous oxide and lidocaine pretreatment has been shown to reduce withdrawal movements during procedures requiring rocuronium injection in pediatric patients. While this study primarily focused on lidocaine, it highlights the potential role of LNO as an adjunct in pain management strategies due to its favorable metabolic profile and reduced toxicity .
Case Studies and Research Findings
- Prophylactic Use Against Sudden Cardiac Death : A patent described the use of LNO to prevent sudden cardiac death in high-risk patients. The findings indicated that LNO could be safely administered prior to inducing ventricular fibrillation without compromising cardiac function .
- Reduction of Toxic Metabolites : In a comparative study involving rats treated with lidocaine versus those treated with LNO, it was found that the latter resulted in significantly lower levels of toxic metabolites such as DMA. This suggests that LNO not only acts as a safer alternative but may also improve patient outcomes by minimizing side effects associated with traditional lidocaine therapy .
Q & A
Basic Research Questions
Q. What are the recommended methods for synthesizing and characterizing Lidocaine N-oxide in laboratory settings?
this compound synthesis typically involves oxidation of lidocaine using oxidizing agents like meta-chloroperbenzoic acid (mCPBA). Key steps include:
- Reaction conditions : Controlled pH and temperature (e.g., ice bath for exothermic reactions) to optimize yield .
- Purification : Column chromatography or recrystallization using solvents like methanol or chloroform, guided by solubility data .
- Characterization : Nuclear magnetic resonance (NMR) for structural confirmation, mass spectrometry (MS) for molecular weight validation, and infrared (IR) spectroscopy to identify functional groups (e.g., N-oxide stretch at ~1250 cm⁻¹) .
- Purity validation : High-performance liquid chromatography (HPLC) with UV detection and comparison to reference standards .
Q. What analytical techniques are validated for detecting this compound in environmental or biological matrices?
- Liquid chromatography-tandem mass spectrometry (LC-MS/MS) is preferred for trace-level detection in environmental samples (e.g., water), with solid-phase extraction (SPE) to minimize matrix interference .
- Sample preparation : Adjust pH to enhance stability; use deuterated internal standards (e.g., this compound-d₃) to correct for recovery losses .
- Quality controls : Include blanks, spikes, and replicates to validate limits of detection (LOD) and quantification (LOQ) .
Advanced Research Questions
Q. How can researchers investigate the transport mechanisms of this compound in cellular models, and what methodological challenges arise in distinguishing active uptake from passive diffusion?
- Experimental design :
- Use in vitro models like HepG2 or HEK293 cells, with/without transporter overexpression (e.g., OCT1) .
- Compare uptake kinetics in wild-type vs. transporter-knockout models (e.g., CRISPR-edited cells) .
- Controls : Include competitive inhibitors (e.g., tetraethylammonium for OCT1) and temperature-dependent assays (4°C vs. 37°C) to differentiate passive vs. active transport .
- Data interpretation : Normalize results to protein content and validate with siRNA silencing. Contradictory data (e.g., uptake in transporter-negative cells) may indicate alternative pathways .
Q. What experimental models are appropriate for assessing the antimutagenic potential of this compound, and how can researchers control for metabolic interference?
- Ames test : Use Salmonella typhimurium strains (TA98, TA100) with/without metabolic activation (S9 liver fractions) to evaluate mutagenicity and antimutagenicity against known mutagens (e.g., 2-aminoanthracene) .
- Controls : Include positive controls (mutagens alone) and negative controls (solvent-only). Pre-incubate this compound with S9 fractions to assess metabolic stability .
- Statistical analysis : Apply fluctuation tests and Poisson regression to quantify mutation rates; address variability with triplicate assays .
Q. When conflicting data emerge regarding the stability of this compound under varying pH conditions, what analytical strategies can resolve discrepancies?
- Stress testing : Expose the compound to pH 1–13 buffers at 37°C and monitor degradation via HPLC at timed intervals .
- Degradation product identification : Use high-resolution MS (HRMS) and NMR to characterize byproducts (e.g., lidocaine or hydroxylamine derivatives) .
- Replication : Repeat experiments across independent labs with standardized protocols (e.g., ICH Q1A guidelines) to isolate procedural vs. intrinsic variability .
Q. How can in vitro and in vivo models be integrated to study the metabolic pathways of this compound, and what are the critical considerations in cross-species pharmacokinetic extrapolation?
- In vitro models : Liver microsomes or hepatocytes from multiple species (human, rat) to identify cytochrome P450 (CYP) isoforms involved in metabolism .
- In vivo validation : Administer radiolabeled this compound to Oct1-knockout mice and compare plasma/tissue concentrations to wild-type using scintillation counting .
- Species differences : Adjust dose scaling based on body surface area and account for interspecific variation in enzyme expression .
Q. Data Contradiction and Reproducibility
Q. How should researchers address inconsistencies in reported solubility values for this compound across studies?
- Standardize protocols : Use the same solvent grades (e.g., HPLC-grade methanol), saturation methods (e.g., shake-flask), and temperature controls .
- Cross-validate : Compare results with alternative techniques (e.g., gravimetric vs. spectrophotometric quantification) .
- Report metadata : Detail batch-to-batch variability in synthesis and purity levels (e.g., ≥95% by HPLC) .
Q. What strategies ensure methodological reproducibility in studies investigating this compound’s interactions with plasma proteins?
- Equilibrium dialysis : Use membranes with defined molecular weight cutoffs and validate protein binding via ultrafiltration-LC/MS .
- Documentation : Provide exact buffer compositions (e.g., 0.1 M phosphate, pH 7.4) and incubation times in supplementary materials .
- Data sharing : Deposit raw chromatograms and binding isotherms in public repositories (e.g., Zenodo) for independent verification .
Properties
IUPAC Name |
2-(2,6-dimethylanilino)-N,N-diethyl-2-oxoethanamine oxide | |
---|---|---|
Source | PubChem | |
URL | https://pubchem.ncbi.nlm.nih.gov | |
Description | Data deposited in or computed by PubChem | |
InChI |
InChI=1S/C14H22N2O2/c1-5-16(18,6-2)10-13(17)15-14-11(3)8-7-9-12(14)4/h7-9H,5-6,10H2,1-4H3,(H,15,17) | |
Source | PubChem | |
URL | https://pubchem.ncbi.nlm.nih.gov | |
Description | Data deposited in or computed by PubChem | |
InChI Key |
YDVXPJXUHRROBA-UHFFFAOYSA-N | |
Source | PubChem | |
URL | https://pubchem.ncbi.nlm.nih.gov | |
Description | Data deposited in or computed by PubChem | |
Canonical SMILES |
CC[N+](CC)(CC(=O)NC1=C(C=CC=C1C)C)[O-] | |
Source | PubChem | |
URL | https://pubchem.ncbi.nlm.nih.gov | |
Description | Data deposited in or computed by PubChem | |
Molecular Formula |
C14H22N2O2 | |
Source | PubChem | |
URL | https://pubchem.ncbi.nlm.nih.gov | |
Description | Data deposited in or computed by PubChem | |
DSSTOX Substance ID |
DTXSID30183274 | |
Record name | Lignocaine N-oxide | |
Source | EPA DSSTox | |
URL | https://comptox.epa.gov/dashboard/DTXSID30183274 | |
Description | DSSTox provides a high quality public chemistry resource for supporting improved predictive toxicology. | |
Molecular Weight |
250.34 g/mol | |
Source | PubChem | |
URL | https://pubchem.ncbi.nlm.nih.gov | |
Description | Data deposited in or computed by PubChem | |
CAS No. |
2903-45-9 | |
Record name | Lignocaine N-oxide | |
Source | ChemIDplus | |
URL | https://pubchem.ncbi.nlm.nih.gov/substance/?source=chemidplus&sourceid=0002903459 | |
Description | ChemIDplus is a free, web search system that provides access to the structure and nomenclature authority files used for the identification of chemical substances cited in National Library of Medicine (NLM) databases, including the TOXNET system. | |
Record name | Lignocaine N-oxide | |
Source | EPA DSSTox | |
URL | https://comptox.epa.gov/dashboard/DTXSID30183274 | |
Description | DSSTox provides a high quality public chemistry resource for supporting improved predictive toxicology. | |
Record name | LIDOCAINE N2-OXIDE | |
Source | FDA Global Substance Registration System (GSRS) | |
URL | https://gsrs.ncats.nih.gov/ginas/app/beta/substances/25797M378Y | |
Description | The FDA Global Substance Registration System (GSRS) enables the efficient and accurate exchange of information on what substances are in regulated products. Instead of relying on names, which vary across regulatory domains, countries, and regions, the GSRS knowledge base makes it possible for substances to be defined by standardized, scientific descriptions. | |
Explanation | Unless otherwise noted, the contents of the FDA website (www.fda.gov), both text and graphics, are not copyrighted. They are in the public domain and may be republished, reprinted and otherwise used freely by anyone without the need to obtain permission from FDA. Credit to the U.S. Food and Drug Administration as the source is appreciated but not required. | |
Retrosynthesis Analysis
AI-Powered Synthesis Planning: Our tool employs the Template_relevance Pistachio, Template_relevance Bkms_metabolic, Template_relevance Pistachio_ringbreaker, Template_relevance Reaxys, Template_relevance Reaxys_biocatalysis model, leveraging a vast database of chemical reactions to predict feasible synthetic routes.
One-Step Synthesis Focus: Specifically designed for one-step synthesis, it provides concise and direct routes for your target compounds, streamlining the synthesis process.
Accurate Predictions: Utilizing the extensive PISTACHIO, BKMS_METABOLIC, PISTACHIO_RINGBREAKER, REAXYS, REAXYS_BIOCATALYSIS database, our tool offers high-accuracy predictions, reflecting the latest in chemical research and data.
Strategy Settings
Precursor scoring | Relevance Heuristic |
---|---|
Min. plausibility | 0.01 |
Model | Template_relevance |
Template Set | Pistachio/Bkms_metabolic/Pistachio_ringbreaker/Reaxys/Reaxys_biocatalysis |
Top-N result to add to graph | 6 |
Feasible Synthetic Routes
Disclaimer and Information on In-Vitro Research Products
Please be aware that all articles and product information presented on BenchChem are intended solely for informational purposes. The products available for purchase on BenchChem are specifically designed for in-vitro studies, which are conducted outside of living organisms. In-vitro studies, derived from the Latin term "in glass," involve experiments performed in controlled laboratory settings using cells or tissues. It is important to note that these products are not categorized as medicines or drugs, and they have not received approval from the FDA for the prevention, treatment, or cure of any medical condition, ailment, or disease. We must emphasize that any form of bodily introduction of these products into humans or animals is strictly prohibited by law. It is essential to adhere to these guidelines to ensure compliance with legal and ethical standards in research and experimentation.