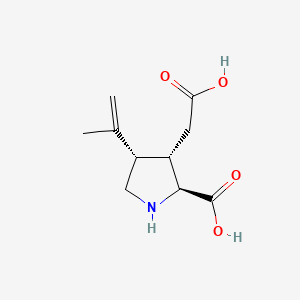
Kainic acid
Overview
Description
Kainic acid (KA) is a naturally occurring excitatory amino acid derived from marine red algae, notably Digenea simplex. It is a potent agonist of ionotropic glutamate receptors, specifically kainate receptors (KARs), and exhibits neuroexcitatory and neurotoxic properties. Structurally, KA features a pyrrolidine dicarboxylate scaffold with an isopropenyl side chain at C-4, distinguishing it from other glutamate analogs . It is widely used in experimental models to study epilepsy, neurodegeneration, and synaptic receptor dynamics due to its ability to induce excitotoxicity and seizures .
Preparation Methods
Classical Synthesis Approaches
Early Isolation and Structural Elucidation
Kainic acid was first isolated in 1953 from the red alga Digenea simplex, where it served as a traditional anthelmintic agent. Initial structural studies via X-ray crystallography revealed its unique (2S,3S,4R) configuration, motivating efforts to replicate its architecture synthetically. Early routes relied on functionalization of pyrrolidine precursors but suffered from low stereocontrol and multi-step sequences. For example, the 1970s synthesis by Oppolzer utilized a chiral camphor-derived auxiliary to establish the C3 and C4 stereocenters, achieving a 7% overall yield after 18 steps.
Proline-Based Syntheses
4-Hydroxy-L-proline emerged as a favored starting material due to its preexisting pyrrolidine ring and stereochemical compatibility. A 2005 route by Parsons et al. converted 4-hydroxy-L-proline into a 3,4-unsaturated pyrrolidine derivative, which underwent high-pressure (15 kbar) Diels-Alder cycloaddition with Danishefsky’s diene. This method achieved a 96% conversion rate but required arduous decarboxylation and oxidation steps, resulting in a 10% final yield.
Modern Stereoselective Methods
Iodolactone Alkylation Strategy
A breakthrough in practicality came from the stereoselective alkylation of iodolactones, as demonstrated in a 2011 synthesis starting from (+)-carvone (Figure 1). The sequence involved:
- Lactonization : Oxidation of (+)-carvone to a bicyclic iodolactone intermediate.
- Alkylation : Stereoselective introduction of the propenyl side chain using Grignard reagents.
- Nitrile Hydrolysis : Conversion of a cyano group to the carboxylic acid under acidic conditions, accompanied by epimerization to correct the C4 configuration.
This route produced 14.6 g of (−)-kainic acid with >98% enantiomeric excess (ee), marking the first gram-scale synthesis.
Method | Starting Material | Key Steps | Yield | Reference |
---|---|---|---|---|
Iodolactone Alkylation | (+)-Carvone | Lactonization, Alkylation, Hydrolysis | 14.6 g |
Ireland-Claisen Rearrangement
The Ireland-Claisen rearrangement enabled efficient construction of the C3–C4 bond. In a 2020 approach, L-tartaric acid was converted to a γ,δ-unsaturated ester, which underwent rearrangement with lithium hexamethyldisilazide (LiHMDS) to establish the stereochemistry. Subsequent palladium-mediated cyclization formed the pyrrolidine ring, yielding this compound in 16.8% overall yield.
Cycloaddition Strategies
High-Pressure Diels-Alder Reaction
Cycloaddition methods exploit the inherent reactivity of dienes and dienophiles to build the bicyclic framework. A notable example used a 3,4-unsaturated pyrrolidine derivative in a high-pressure (15 kbar) Diels-Alder reaction with Danishefsky’s diene, achieving a 96% conversion rate. However, the necessity for specialized equipment limited scalability.
Catalytic Asymmetric Cyclization
Recent advances in catalysis have enabled enantioselective pyrrolidine formation. A 2019 method employed a copper(II) complex with a tert-butyl salicylaldimine ligand to catalyze a [3+2] cycloaddition between an azomethine ylide and methyl acrylate. This single-step process achieved 88% ee and 75% yield, though subsequent oxidation steps were required to install the carboxylic acid.
Biosynthetic and Industrial-Scale Approaches
Biosynthetic Pathways
Biosynthetic studies revealed that this compound originates from L-glutamate and dimethylallyl pyrophosphate (DMAPP) in Digenea simplex. The pathway involves:
- Prenylation : DMAPP transfer to L-glutamate by a prenyltransferase.
- Cyclization : Spontaneous formation of the pyrrolidine ring.
- Oxidation : Hydroxylation and oxidation to the final structure.
Efforts to replicate this pathway in E. coli via metabolic engineering remain ongoing.
Industrial Production
The stereoselective iodolactone alkylation method has become the industrial standard due to its scalability. Key optimizations include:
- Catalyst Recycling : Reuse of Grignard reagents to reduce costs.
- Continuous Flow Hydrolysis : Implementation of flow chemistry for safer nitrile hydrolysis.
Current production costs approximate $50/g, a 95% reduction from pre-2010 levels.
Comparative Analysis of Synthetic Routes
The table below evaluates critical parameters of prominent methods:
Method | Steps | Overall Yield | Stereoselectivity | Scalability |
---|---|---|---|---|
Iodolactone Alkylation | 12 | 23% | >98% ee | Industrial |
High-Pressure Diels-Alder | 18 | 10% | 92% de | Laboratory |
Ireland-Claisen | 15 | 16.8% | 95% de | Pilot Plant |
Catalytic Cycloaddition | 9 | 34% | 88% ee | Laboratory |
Chemical Reactions Analysis
Types of Reactions: Kainic acid undergoes various chemical reactions, including oxidation, reduction, and substitution reactions. It is known to participate in oxidative cyclization reactions, where intramolecular carbon-carbon bond formation occurs . Additionally, this compound can be radiolabeled through tritiation, which involves the exchange of hydrogen atoms with tritium using specific catalysts .
Common Reagents and Conditions: Common reagents used in the synthesis and modification of this compound include rhodium on alumina, tritiated water, and Wittig reagents . The reaction conditions often involve heating at elevated temperatures and the use of specific catalysts to achieve the desired transformations .
Major Products: The major products formed from the reactions of this compound include its radiolabeled derivatives, which are used in various research applications . These derivatives are valuable tools for studying the pharmacokinetics and distribution of this compound in biological systems .
Scientific Research Applications
Modeling Epilepsy
Kainic acid is extensively used to induce seizures in laboratory animals, particularly in studies focused on temporal lobe epilepsy (TLE). The intra-amygdala injection of this compound has been shown to replicate key features of human drug-resistant TLE, making it a critical model for understanding seizure mechanisms and testing potential antiepileptic drugs .
Table 1: this compound Dose and Effects on Seizure Models
Animal Model | Route of Administration | Typical Dose (mg/kg) | Observed Effects |
---|---|---|---|
Mice | Intra-amygdala | 10-30 | Induction of seizures |
Rats | Systemic Injection | 15-25 | Neuronal excitotoxicity |
Sprague-Dawley | Intra-hippocampal | 20 | Cell death in hippocampal neurons |
Investigating Neurodegenerative Diseases
This compound-induced excitotoxicity serves as a model for studying several neurodegenerative diseases, including Alzheimer's disease, Huntington's disease, and amyotrophic lateral sclerosis (ALS). Research has demonstrated that this compound can induce cellular changes similar to those observed in these conditions, such as oxidative stress and inflammation .
Case Study: this compound and Alzheimer's Disease
Mechanism of Action
Kainic acid exerts its effects by acting as an agonist for kainate receptors, a subtype of ionotropic glutamate receptors . When this compound binds to these receptors, it induces the opening of sodium channels, leading to an influx of sodium ions and subsequent depolarization of the neuron . This depolarization triggers excitatory postsynaptic potentials and can result in neuronal excitation and, in high concentrations, excitotoxicity .
The activation of kainate receptors by this compound also leads to the release of other neurotransmitters, such as glutamate, which further amplifies the excitatory signals . The overstimulation of neurons can cause calcium influx, mitochondrial dysfunction, and the generation of reactive oxygen species, ultimately leading to neuronal damage and death .
Comparison with Similar Compounds
Comparison with Structural Analogs
Kainic acid belongs to the kainoid family, which includes domoic acid, isodomoic acids, and synthetic derivatives. Key structural and functional differences are outlined below:
Table 1: Structural and Functional Comparison of this compound and Related Compounds
Key Insights :
- Domoic acid exhibits higher receptor affinity and toxicity than KA, causing severe neurological effects in humans .
- Isodomoic acids and synthetic derivatives (e.g., dihydrothis compound) are used to probe structure-activity relationships (SAR) of KARs .
- β-Kainic acid , a stereoisomer, shows diminished receptor activation, highlighting the importance of stereochemistry in ligand-receptor interactions .
Functional Analogs and Receptor Interactions
KA shares functional similarities with other glutamate receptor agonists, though its selectivity for KARs distinguishes it from AMPA or NMDA receptor-targeting compounds:
Table 2: Functional Comparison with Non-Kainoid Agonists
Key Insights :
- Acromelic Acid A , isolated from mushrooms, surpasses KA in neurotoxic potency (EC50 = 2.5 μM vs. 70 μM for KA) .
- Quisqualic acid activates AMPA receptors more strongly, making KA a more selective tool for KAR studies .
Neurotoxic Mechanisms and Comparative Studies
KA’s neurotoxicity is linked to sustained calcium influx and oxidative stress, a mechanism shared with domoic acid but distinct from AMPA receptor agonists:
- Calcium Dynamics : KA induces a biphasic calcium influx in neurons: a rapid transient phase (threshold ≥12.5 μM) and a sustained phase (≥25 μM) correlating with neurodegeneration .
- Oxidative Stress: KA-mediated excitotoxicity generates reactive oxygen species (ROS), exacerbating hippocampal damage, a feature less pronounced in domoic acid models .
Table 3: Neurotoxic Outcomes in Rodent Models
Biological Activity
Kainic acid (KA) is a potent excitatory amino acid that acts primarily as an agonist for the kainate subtype of glutamate receptors. Its biological activity has been extensively studied, particularly in the context of neurodegeneration, epilepsy, and excitotoxicity. This article reviews the mechanisms of action, experimental models, and implications of KA in various biological contexts, supported by data tables and relevant case studies.
This compound induces excitotoxicity primarily through the activation of ionotropic glutamate receptors, leading to increased intracellular calcium levels. This process triggers a cascade of events resulting in neuronal apoptosis and inflammation. Key mechanisms include:
- Neuronal Excitation : KA binds to AMPA/kainate receptors, causing depolarization and subsequent calcium influx into neurons .
- Oxidative Stress : Increased calcium levels lead to the production of reactive oxygen species (ROS), contributing to mitochondrial dysfunction and cell death .
- Inflammatory Response : Systemic administration of KA activates glial cells, mimicking inflammatory responses observed in neurodegenerative diseases .
Experimental Models
This compound is widely used in animal models to study various neurological conditions, particularly temporal lobe epilepsy (TLE). Different administration routes (systemic, intrahippocampal, intranasal) yield varying effects on seizure severity and neuronal damage:
Case Study: N-Shc Signaling Pathway
Recent research has explored the role of the N-Shc signaling pathway in KA-induced seizures. In a study involving N-Shc knockout mice, it was found that these mice exhibited significantly reduced seizure severity and neuronal loss compared to wild-type controls. The results are summarized in the table below:
Mouse Strain | Maximum Seizure Score | Duration of Seizures (min) |
---|---|---|
C57BL/6 | 5.21 ± 0.98 | 167.2 ± 63.8 |
N-Shc+/+ | 5.50 ± 0.71 | 172.1 ± 24.6 |
N-Shc−/− | 3.27 ± 1.78 | 95.3 ± 32.6 |
The reduced seizure activity in N-Shc−/− mice suggests that targeting this pathway could be a potential therapeutic strategy for managing KA-induced excitotoxicity .
Neurodegenerative Implications
This compound's role in neurodegenerative diseases has been highlighted through various studies indicating its ability to model conditions such as Alzheimer's disease:
- Amyloid Precursor Protein Processing : KA administration alters the processing of amyloid precursor protein, potentially influencing amyloid plaque formation .
- Tau Protein Expression : Changes in tau protein expression have been observed following KA treatment, linking it to neurofibrillary tangles seen in Alzheimer's pathology .
Metabolomics Profiling
A metabolomics study on rats subjected to KA revealed significant metabolic changes in both plasma and hippocampus at different time points post-treatment:
Q & A
Basic Research Questions
Q. Q1. What experimental models utilize kainic acid to study excitotoxicity and seizure mechanisms?
KA is widely used to model temporal lobe epilepsy and neurodegeneration. The intrahippocampal KA (IHKA) model in rodents involves stereotaxic injection into the hippocampus to induce status epilepticus (SE), followed by chronic epilepsy development (Figure S1, ). Key endpoints include EEG monitoring for seizure activity, histological analysis of neuronal loss (e.g., NeuN staining), and glial activation (GFAP/Iba1) . Methodological rigor requires strain selection (e.g., FVB/N vs. C57BL/6 mice show differential KA sensitivity ) and standardized SE duration quantification .
Q. Q2. How does KA interact with glutamate receptors to induce neurotoxicity?
KA binds to ionotropic kainate receptors (GluK1-5), causing prolonged depolarization, Ca²⁺ influx, and oxidative stress. Advanced studies use radioligand binding assays (e.g., [³H]-KA displacement by acromelic acid A in rat synaptic membranes) to quantify receptor affinity (Ki = 15.1 nM and 1.49 pM for high/low-affinity sites) and competitive inhibition by analogs like domoic acid . Thiocyanate inclusion in assays modulates AMPA receptor binding, revealing KA’s dual receptor interactions .
Q. Q3. What are the natural sources of KA, and how do concentrations vary?
KA is naturally produced in the red seaweed Palmaria palmata (dulse). Concentrations range from trace levels to 560 µg/g dry weight (dw) in European commercial samples, with extreme variability (e.g., 10,000 µg/g dw in mutant strains ). Methodological challenges include sample preparation (freeze-drying preserves KA ) and HPLC/MS validation to distinguish KA from analogs like 1-hydroxythis compound .
Advanced Research Questions
Q. Q4. How can contradictions in reported KA concentrations in P. palmata be resolved?
Discrepancies arise from strain differences, environmental factors, and analytical methods. Ramsey et al. (1994) reported 4,000–10,000 µg/g dw in mutant strains , while recent studies show <560 µg/g dw in commercial samples . Researchers must standardize protocols: (1) quantify residual moisture to express results as µg/g dw , (2) validate extraction efficiency (e.g., acidified methanol), and (3) screen for geographical/seasonal variability .
Q. Q5. What genetic factors influence KA-induced neuronal susceptibility in animal models?
Mouse strain differences (e.g., FVB/N vs. C57BL/6) significantly affect KA sensitivity due to intrinsic neuronal properties and extrinsic glial responses. Chimeric studies show FVB/N neurons in C57BL/6 hosts retain vulnerability, implicating cell-autonomous mechanisms (e.g., antioxidant capacity) . Transcriptomic profiling of hippocampal subregions (CA1 vs. DG) post-KA exposure can identify candidate genes (e.g., Arc and c-fos activation ).
Q. Q6. How can KA biosynthesis be optimized for scalable research use?
Traditional extraction from seaweed is inefficient. UC San Diego’s bioengineering approach uses biocatalysis (seaweed-derived enzymes) and bacterial fermentation to produce gram-scale KA with >90% purity. This method reduces reagent costs by 90% vs. chemical synthesis and simplifies purification . Critical parameters include enzyme kinetics optimization and strain engineering for yield improvement.
Q. Q7. What pharmacological interventions mitigate KA-induced neurotoxicity, and how are they validated?
Anticonvulsant candidates (e.g., β-hydroxybutyrate [BHB], trans-caryophyllene [TC]) are tested in KA rodent models. Key steps:
- Dose optimization : BHB (100 mg/kg, i.p.) reduces SE duration and mortality .
- Biomarker analysis : TC lowers malondialdehyde (oxidative stress) and TNF-α/IL-1β (neuroinflammation) .
- Behavioral scoring : Seizure severity scales (e.g., Racine stages) must be blinded to treatment groups .
Q. Methodological Best Practices
Experimental Design
- Controls : Include PBS-injected sham groups to distinguish KA-specific effects .
- Power analysis : For neurohistology, n ≥ 8/group reduces variability from strain differences .
- Temporal resolution : Profile acute (1–24 hr) vs. chronic (weeks) phases post-KA .
Data Interpretation
Properties
IUPAC Name |
(2S,3S,4S)-3-(carboxymethyl)-4-prop-1-en-2-ylpyrrolidine-2-carboxylic acid | |
---|---|---|
Source | PubChem | |
URL | https://pubchem.ncbi.nlm.nih.gov | |
Description | Data deposited in or computed by PubChem | |
InChI |
InChI=1S/C10H15NO4/c1-5(2)7-4-11-9(10(14)15)6(7)3-8(12)13/h6-7,9,11H,1,3-4H2,2H3,(H,12,13)(H,14,15)/t6-,7+,9-/m0/s1 | |
Source | PubChem | |
URL | https://pubchem.ncbi.nlm.nih.gov | |
Description | Data deposited in or computed by PubChem | |
InChI Key |
VLSMHEGGTFMBBZ-OOZYFLPDSA-N | |
Source | PubChem | |
URL | https://pubchem.ncbi.nlm.nih.gov | |
Description | Data deposited in or computed by PubChem | |
Canonical SMILES |
CC(=C)C1CNC(C1CC(=O)O)C(=O)O | |
Source | PubChem | |
URL | https://pubchem.ncbi.nlm.nih.gov | |
Description | Data deposited in or computed by PubChem | |
Isomeric SMILES |
CC(=C)[C@H]1CN[C@@H]([C@H]1CC(=O)O)C(=O)O | |
Source | PubChem | |
URL | https://pubchem.ncbi.nlm.nih.gov | |
Description | Data deposited in or computed by PubChem | |
Molecular Formula |
C10H15NO4 | |
Source | PubChem | |
URL | https://pubchem.ncbi.nlm.nih.gov | |
Description | Data deposited in or computed by PubChem | |
DSSTOX Substance ID |
DTXSID7040526 | |
Record name | Kainic acid | |
Source | EPA DSSTox | |
URL | https://comptox.epa.gov/dashboard/DTXSID7040526 | |
Description | DSSTox provides a high quality public chemistry resource for supporting improved predictive toxicology. | |
Molecular Weight |
213.23 g/mol | |
Source | PubChem | |
URL | https://pubchem.ncbi.nlm.nih.gov | |
Description | Data deposited in or computed by PubChem | |
CAS No. |
487-79-6, 59905-23-6 | |
Record name | α-Kainic acid | |
Source | CAS Common Chemistry | |
URL | https://commonchemistry.cas.org/detail?cas_rn=487-79-6 | |
Description | CAS Common Chemistry is an open community resource for accessing chemical information. Nearly 500,000 chemical substances from CAS REGISTRY cover areas of community interest, including common and frequently regulated chemicals, and those relevant to high school and undergraduate chemistry classes. This chemical information, curated by our expert scientists, is provided in alignment with our mission as a division of the American Chemical Society. | |
Explanation | The data from CAS Common Chemistry is provided under a CC-BY-NC 4.0 license, unless otherwise stated. | |
Record name | Kainic acid [INN:JAN] | |
Source | ChemIDplus | |
URL | https://pubchem.ncbi.nlm.nih.gov/substance/?source=chemidplus&sourceid=0000487796 | |
Description | ChemIDplus is a free, web search system that provides access to the structure and nomenclature authority files used for the identification of chemical substances cited in National Library of Medicine (NLM) databases, including the TOXNET system. | |
Record name | kainic acid | |
Source | DTP/NCI | |
URL | https://dtp.cancer.gov/dtpstandard/servlet/dwindex?searchtype=NSC&outputformat=html&searchlist=136038 | |
Description | The NCI Development Therapeutics Program (DTP) provides services and resources to the academic and private-sector research communities worldwide to facilitate the discovery and development of new cancer therapeutic agents. | |
Explanation | Unless otherwise indicated, all text within NCI products is free of copyright and may be reused without our permission. Credit the National Cancer Institute as the source. | |
Record name | Kainic acid | |
Source | EPA DSSTox | |
URL | https://comptox.epa.gov/dashboard/DTXSID7040526 | |
Description | DSSTox provides a high quality public chemistry resource for supporting improved predictive toxicology. | |
Record name | KAINIC ACID | |
Source | FDA Global Substance Registration System (GSRS) | |
URL | https://gsrs.ncats.nih.gov/ginas/app/beta/substances/SIV03811UC | |
Description | The FDA Global Substance Registration System (GSRS) enables the efficient and accurate exchange of information on what substances are in regulated products. Instead of relying on names, which vary across regulatory domains, countries, and regions, the GSRS knowledge base makes it possible for substances to be defined by standardized, scientific descriptions. | |
Explanation | Unless otherwise noted, the contents of the FDA website (www.fda.gov), both text and graphics, are not copyrighted. They are in the public domain and may be republished, reprinted and otherwise used freely by anyone without the need to obtain permission from FDA. Credit to the U.S. Food and Drug Administration as the source is appreciated but not required. | |
Retrosynthesis Analysis
AI-Powered Synthesis Planning: Our tool employs the Template_relevance Pistachio, Template_relevance Bkms_metabolic, Template_relevance Pistachio_ringbreaker, Template_relevance Reaxys, Template_relevance Reaxys_biocatalysis model, leveraging a vast database of chemical reactions to predict feasible synthetic routes.
One-Step Synthesis Focus: Specifically designed for one-step synthesis, it provides concise and direct routes for your target compounds, streamlining the synthesis process.
Accurate Predictions: Utilizing the extensive PISTACHIO, BKMS_METABOLIC, PISTACHIO_RINGBREAKER, REAXYS, REAXYS_BIOCATALYSIS database, our tool offers high-accuracy predictions, reflecting the latest in chemical research and data.
Strategy Settings
Precursor scoring | Relevance Heuristic |
---|---|
Min. plausibility | 0.01 |
Model | Template_relevance |
Template Set | Pistachio/Bkms_metabolic/Pistachio_ringbreaker/Reaxys/Reaxys_biocatalysis |
Top-N result to add to graph | 6 |
Feasible Synthetic Routes
Disclaimer and Information on In-Vitro Research Products
Please be aware that all articles and product information presented on BenchChem are intended solely for informational purposes. The products available for purchase on BenchChem are specifically designed for in-vitro studies, which are conducted outside of living organisms. In-vitro studies, derived from the Latin term "in glass," involve experiments performed in controlled laboratory settings using cells or tissues. It is important to note that these products are not categorized as medicines or drugs, and they have not received approval from the FDA for the prevention, treatment, or cure of any medical condition, ailment, or disease. We must emphasize that any form of bodily introduction of these products into humans or animals is strictly prohibited by law. It is essential to adhere to these guidelines to ensure compliance with legal and ethical standards in research and experimentation.