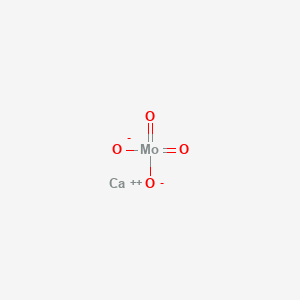
Calcium molybdate
- Click on QUICK INQUIRY to receive a quote from our team of experts.
- With the quality product at a COMPETITIVE price, you can focus more on your research.
Overview
Description
Calcium molybdate (CaMoO₄) is an inorganic compound with a tetragonal scheelite-type crystal structure (space group I41/a) . It occurs naturally as the mineral powellite and is synthesized for applications in catalysis, electrochemistry, optoelectronics, scintillation detectors, and nuclear waste management . Its high thermal stability, radiation resistance, and tunable optical properties (e.g., bandgap energy ~3.96 eV for pure CaMoO₄) make it versatile . Nanoparticles (NPs) of CaMoO₄ can be synthesized via methods such as coprecipitation, hydrothermal synthesis, and inverse miniemulsion, achieving particle sizes as small as 12 nm .
Preparation Methods
Precipitation-Based Synthesis
Precipitation remains the most widely used method for calcium molybdate synthesis due to its simplicity and scalability. The process typically involves reacting aqueous solutions of calcium salts with molybdate precursors under controlled pH conditions. For instance, early industrial methods described in patents utilized milk of lime (calcium hydroxide suspension) to precipitate this compound from sodium molybdate solutions. The reaction follows:
$$
\text{Ca(OH)}2 + \text{Na}2\text{MoO}4 \rightarrow \text{CaMoO}4 \downarrow + 2\text{NaOH}
$$
Key parameters include reactant concentration, pH (optimized between 9–11), and stirring rate. Higher pH values favor the formation of crystalline CaMoO₄ by suppressing the hydrolysis of molybdate ions into polymeric species. However, this method often yields micron-sized particles with irregular morphologies, necessitating post-synthesis annealing to enhance crystallinity.
Hydrothermal and Solvothermal Methods
Hydrothermal synthesis enables precise control over particle size and morphology by leveraging high-temperature aqueous or solvent-based reactions in sealed autoclaves. A notable advancement is the rapid-injection technique, where preheated calcium and molybdate solutions are mixed abruptly, inducing rapid nucleation. For example, Jie Song et al. demonstrated that injecting 0.1 M calcium nitrate into a 0.1 M sodium molybdate solution at 100°C produces doughnut-shaped CaMoO₄ microstructures with an average diameter of 2–5 μm. Adjusting the water-to-ethylene glycol ratio in the solvent further modulates aspect ratios, achieving spindle-like or sheet-like morphologies.
Role of Solvent Composition
Binary solvent systems (e.g., water/ethylene glycol) reduce interfacial tension, promoting anisotropic growth. Ethylene glycol’s high viscosity slows diffusion rates, favoring the formation of hierarchical structures. X-ray diffraction (XRD) analysis confirms that products synthesized in glycol-rich solvents exhibit enhanced crystallinity with a tetragonal scheelite structure (space group I4₁/a).
Combustion Synthesis
Combustion synthesis is a high-energy, exothermic process suitable for producing nano-crystalline this compound. Using fuels like glycine or urea, this method involves heating precursor mixtures to 300–500°C, resulting in rapid oxide formation. A study by Gaochao Fan et al. revealed that combustion at 500°C yields CaMoO₄ nanoparticles (20–50 nm) with high phase purity, as evidenced by sharp XRD peaks corresponding to the (112) and (204) planes.
Temperature-Dependent Phase Evolution
Molecular dynamics simulations correlate increased synthesis temperatures with improved crystallinity and reduced lattice strain. Combustion at 500°C produces materials with a Brunauer-Emmett-Teller (BET) surface area of 15 m²/g, ideal for electrochemical applications. Electrochemical impedance spectroscopy (EIS) further shows that higher crystallinity reduces charge-transfer resistance, enhancing energy storage performance.
Inverse Miniemulsion (ME) Synthesis
The inverse miniemulsion technique confines reactants within nanoscale water-in-oil droplets, enabling precise control over particle size and dopant distribution. In a recent study, calcium nitrate and sodium molybdate solutions were dispersed in a cyclohexane/surfactant mixture, resulting in CaMoO₄ nanoparticles (10–15 nm) with narrow size distributions.
Europium Doping and Luminescence
Introducing europium (Eu³⁺) into the ME system achieves homogeneous doping due to spatial confinement. Inductively coupled plasma-mass spectrometry (ICP-MS) confirms that 5 at.% Eu-doped CaMoO₄ exhibits uniform ion distribution, whereas batch methods yield heterogeneous clusters. Photoluminescence (PL) spectra reveal a strong red emission at 612 nm (⁵D₀→⁷F₂ transition) with a quantum efficiency of 68%, surpassing conventional samples by 20%.
Comparative Analysis of Synthesis Methods
Table 1 summarizes the critical parameters and outcomes of this compound preparation techniques:
Method | Temperature | Particle Size | Crystallinity | Key Advantages |
---|---|---|---|---|
Precipitation | 25–100°C | 1–10 μm | Moderate | Low cost, scalable |
Hydrothermal | 100–200°C | 0.5–5 μm | High | Morphology control |
Combustion | 300–500°C | 20–50 nm | Very High | Rapid, energy-efficient |
Inverse Miniemulsion | 25–50°C | 10–15 nm | Moderate | Narrow size distribution, doping control |
Table 2 correlates synthesis conditions with functional properties:
Property | Precipitation | Hydrothermal | Combustion | Miniemulsion |
---|---|---|---|---|
Surface Area (m²/g) | 5–10 | 8–15 | 10–20 | 30–50 |
Photoluminescence | Weak | Moderate | Moderate | Strong |
Electrochemical Capacity | Low | Moderate | High | N/A |
Chemical Reactions Analysis
Reaction with Sodium Carbonate
Calcium molybdate undergoes metathesis with sodium carbonate to form sodium molybdate (Na₂MoO₄) and calcium carbonate (CaCO₃):
CaMoO4+Na2CO3→Na2MoO4+CaCO3↓
Conditions :
-
Countercurrent extraction (4–5 stages) optimizes dissolution and cost efficiency .
-
Excess Na₂CO₃ (~10–15% above stoichiometric) ensures complete precipitation .
Applications :
Nitric Acid Leaching
Reaction with dilute HNO₃ produces molybdic acid (H₂MoO₄) and calcium nitrate:
CaMoO4+2HNO3→H2MoO4↓+Ca NO3 2
Challenges :
Hydrochloric Acid Leaching
HCl leaching avoids insoluble byproducts (e.g., CaSO₄):
CaMoO4+2HCl→H2MoO4↓+CaCl2
Efficiency :
Acid Type | Mo Recovery (%) | Byproduct Handling |
---|---|---|
HNO₃ | 85–90 | Complex filtration |
HCl | >95 | Simplified process |
Precipitation from Calcium Sulfate
Nanoparticle synthesis via ion exchange:
CaSO4+Na2MoO4→CaMoO4↓+Na2SO4
Conditions :
Behavior in Acidic Solutions
In strongly acidic media, CaMoO₄ dissolves and condenses into polymolybdates:
6MoO42−+10H+→Mo6O192−+5H2O
Key Observations :
Thermal Stability
This compound exhibits high thermal stability but decomposes above 600°C:
CaMoO4ΔCaO+MoO3
Data :
Scientific Research Applications
Catalysis and Chemical Reactions
Calcium molybdate is widely utilized as a catalyst in various chemical reactions due to its unique properties. Its ability to facilitate reactions while remaining stable under high temperatures makes it suitable for industrial applications.
- Case Study: Synthesis of this compound Nanoparticles
A study demonstrated the synthesis of this compound nanoparticles from phosphogypsum, a waste product from the fertilizer industry. The nanoparticles exhibited high purity and small particle sizes (12 nm), indicating their potential use in catalysis and other applications .
Optoelectronics
This compound has promising applications in the field of optoelectronics, particularly in solar cells.
- Research Findings
Recent studies explored the use of this compound in solar cell photoanodes, revealing that its optical and electrical properties can be optimized through careful control of synthesis conditions such as pH and temperature. The best-performing samples showed potential for use in photovoltaic devices, achieving notable efficiency metrics .
Corrosion Inhibition
This compound serves as an effective corrosion inhibitor in various environments, particularly in water-based systems.
- Application Insights
Molybdates, including this compound, are used in cooling systems and hydraulic fluids to prevent corrosion of metal components. They work by forming a protective oxide layer on metal surfaces, thereby enhancing durability and longevity .
Pigments and Coatings
Molybdate compounds are often employed as pigments due to their stability and non-toxic nature.
- Usage Overview
This compound is used in paints and coatings as a white pigment and corrosion inhibitor. It is combined with other compounds to produce vibrant colors while providing protective properties against environmental degradation .
Energy Storage
Recent research has investigated the role of this compound in energy storage systems, particularly as a substitute for lithium-based materials.
- Study Findings
This compound has been evaluated for use in sodium-based energy storage devices, showing promise due to its abundance and cost-effectiveness compared to lithium . This research highlights the potential for this compound to contribute to sustainable energy solutions.
Flame Retardants
This compound is also utilized as a flame retardant in various materials.
- Application Context
It is incorporated into cellulosic materials and textiles to enhance fire resistance, making it suitable for use in furniture, draperies, and other upholstery products .
Summary Table of Applications
Mechanism of Action
The mechanism of action of calcium molybdate involves its role as a catalyst in various chemical reactions. The compound’s unique structure and properties enable it to facilitate redox reactions, enhancing the efficiency of these processes. In electrochemical applications, the morphology of this compound provides enhanced ion diffusivity and electron transport, making it suitable for energy storage applications .
Comparison with Similar Compounds
Structural and Crystallographic Properties
Calcium molybdate belongs to the scheelite family (ABO₄), which includes compounds like calcium tungstate (CaWO₄), strontium molybdate (SrMoO₄), and barium molybdate (BaMoO₄). These compounds share a tetragonal structure but differ in lattice parameters and ionic radii of the A-site cation (Ca²⁺, Sr²⁺, Ba²⁺) .
Key Observations :
- Larger A-site cations (e.g., Ba²⁺) increase lattice parameters, reducing bandgap energy due to enhanced electron delocalization .
- CaMoO₄ and CaWO₄ exhibit similar structures but differ in optical properties due to the Mo⁶⁺/W⁶⁺ substitution .
This compound :
- Room-temperature coprecipitation using phosphogypsum waste yields NPs with 12 nm average size and high purity .
- Inverse miniemulsion enables precise control over particle size (10–50 nm) and crystallinity .
- Hydrothermal synthesis produces spherical particles for optoelectronic applications .
Comparisons :
- Strontium/Barium Molybdate : Typically synthesized via solid-state reactions or sol-gel methods, resulting in larger particles (50–200 nm) due to higher thermal stability .
- Calcium Tungstate: Requires high-temperature sintering (>800°C) for single-crystal growth, limiting nanoscale applications .
Optical and Functional Properties
Photoluminescence and Scintillation
- CaMoO₄ : Exhibits green-to-orange emission due to [MoO₄]²⁻ charge transfer transitions. Doping with Eu³⁺ or Nd³⁺ enhances photoluminescence for phosphor applications .
- CaWO₄ : Shows blue emission under UV excitation, widely used in X-ray scintillators .
- SrMoO₄/BaMoO₄ : Lower luminescence efficiency but better thermal quenching resistance .
Bandgap Engineering
Doping CaMoO₄ with rare-earth ions (e.g., Nd³⁺) reduces its bandgap from 3.96 eV to 1.26 eV, enabling visible-light photocatalysis . Similar doping in SrMoO₄ or BaMoO₄ results in less pronounced bandgap shifts due to their inherently lower defect tolerance .
Q & A
Basic Research Questions
Q. What synthesis methods are most effective for producing phase-pure calcium molybdate nanoparticles, and how do synthesis temperatures influence crystallinity?
this compound nanoparticles are synthesized via combustion routes (300–500°C) or microwave-assisted hydrothermal methods. Combustion at 500°C yields highly crystalline CaMoO₄ with tetragonal scheelite structure, confirmed by XRD and Raman spectroscopy . Lower temperatures (300°C) produce smaller crystallites but may introduce amorphous phases, impacting electrochemical performance in energy storage applications . For reproducible synthesis:
- Use stoichiometric precursors (e.g., Ca(NO₃)₂·4H₂O and (NH₄)₆Mo₇O₂₄·4H₂O).
- Optimize calcination time (2–4 hrs) to minimize impurity phases.
- Characterize phase purity via Rietveld refinement .
Q. How can vibrational spectroscopy resolve contradictory assignments of low-frequency modes in CaMoO₄?
Infrared (IR) and Raman studies of CaMoO₄ reveal conflicting assignments for ν₂ (bending) and ν₄ (stretching) modes of MoO₄²⁻ tetrahedra. Methodological solutions include:
- Isotopic substitution (e.g., ⁹⁸Mo vs. ¹⁰⁰Mo) to isolate mode contributions .
- Single-crystal polarized spectroscopy to distinguish between Au (IR-active) and Eu (Raman-active) modes, resolving discrepancies from polycrystalline data .
- Cross-validation with density functional theory (DFT) phonon calculations to match experimental frequencies .
Q. What experimental techniques are critical for determining the thermal expansion coefficients of CaMoO₄?
Synchrotron high-resolution powder diffraction (12–300 K) and lattice dynamics calculations are essential:
Advanced Research Questions
Q. How do intrinsic defects in CaMoO₄ influence its scintillation efficiency, and how can defect formation energies be calculated?
Defects (e.g., oxygen vacancies, cation substitutions) alter charge-carrier trapping and luminescence. For defect analysis:
- Computational method : Use DFT with hybrid functionals (e.g., HSE06) to calculate defect formation energies under different oxygen chemical potentials .
- Key findings : Rare-earth doping (e.g., Eu³+) introduces trap states that enhance radiative recombination, improving scintillation light yield .
- Experimental validation : Thermoluminescence (TL) glow curves and X-ray absorption near-edge spectroscopy (XANES) .
Q. What computational frameworks are optimal for modeling electronic structure and optical properties of CaMoO₄?
- Exchange-correlation functionals : The Perdew-Burke-Ernzerhof (PBE) generalized gradient approximation (GGA) accurately predicts bandgaps (~4.1 eV) but underestimates excitonic effects. Include spin-orbit coupling for Mo 4d-states .
- Plane-wave codes : Use VASP with PAW pseudopotentials and a 500 eV cutoff. Optimize k-point sampling via Monkhorst-Pack grids (e.g., 4×4×4 for bulk calculations) .
- Optical properties : Compute dielectric function (ε₁, ε₂) using the GW-BSE method to match experimental refractive indices (n ≈ 1.9–2.1 at 600 nm) .
Q. How does CaMoO₄ perform as a bolometric material in neutrino-less double-beta decay (0νββ) experiments, and what are key experimental design considerations?
In the AMoRE experiment, CaMoO₄ crystals enriched with ¹⁰⁰Mo and depleted in ⁴⁸Ca are used as scintillating bolometers:
- Key parameters :
- Light yield: 5,200 photons/MeV at 10 mK .
- Radioactive purity: U/Th contamination < 10⁻⁵ g/g .
- Design challenges :
- Mitigate thermal noise via cryogenic shielding (<20 mK).
- Optimize crystal geometry (e.g., 40 mm diameter × 40 mm height) to maximize ¹⁰⁰Mo content while minimizing self-absorption .
Methodological Tables
Contradictions & Resolutions
- Vibrational mode assignments : Conflicting IR/Raman data arise from polycrystalline vs. single-crystal measurements. Resolution requires polarized spectroscopy and isotopic labeling .
- Bandgap discrepancies : Hybrid functionals (e.g., HSE06) correct PBE’s underestimation of Mo 4d-orbital localization .
Properties
CAS No. |
7789-82-4 |
---|---|
Molecular Formula |
CaMoO4-6 |
Molecular Weight |
200.03 g/mol |
IUPAC Name |
calcium;molybdenum;oxygen(2-) |
InChI |
InChI=1S/Ca.Mo.4O/q+2;;4*-2 |
InChI Key |
RKZUBBQQQBQBGT-UHFFFAOYSA-N |
SMILES |
[O-][Mo](=O)(=O)[O-].[Ca+2] |
Canonical SMILES |
[O-2].[O-2].[O-2].[O-2].[Ca+2].[Mo] |
Appearance |
Solid powder |
density |
4.4 g/cm³ |
Key on ui other cas no. |
7789-82-4 |
physical_description |
WHITE CRYSTALLINE POWDER. |
Pictograms |
Irritant; Health Hazard |
Purity |
>98% (or refer to the Certificate of Analysis) |
shelf_life |
>3 years if stored properly |
solubility |
Solubility in water, g/100ml at 25 °C: 0.005 (very poor) |
storage |
Dry, dark and at 0 - 4 C for short term (days to weeks) or -20 C for long term (months to years). |
Synonyms |
Calcium molybdate |
Origin of Product |
United States |
Disclaimer and Information on In-Vitro Research Products
Please be aware that all articles and product information presented on BenchChem are intended solely for informational purposes. The products available for purchase on BenchChem are specifically designed for in-vitro studies, which are conducted outside of living organisms. In-vitro studies, derived from the Latin term "in glass," involve experiments performed in controlled laboratory settings using cells or tissues. It is important to note that these products are not categorized as medicines or drugs, and they have not received approval from the FDA for the prevention, treatment, or cure of any medical condition, ailment, or disease. We must emphasize that any form of bodily introduction of these products into humans or animals is strictly prohibited by law. It is essential to adhere to these guidelines to ensure compliance with legal and ethical standards in research and experimentation.