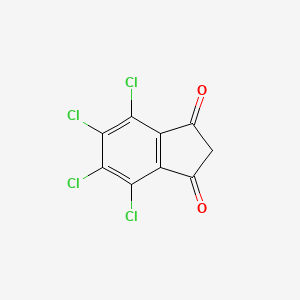
TCID
Overview
Description
TCID (referred to in this context as a small-molecule inhibitor targeting the deubiquitinating enzyme UCHL3) is a preclinical therapeutic agent designed to modulate ubiquitin-dependent signaling pathways. UCHL3, a member of the ubiquitin C-terminal hydrolase family, regulates protein stability by removing ubiquitin moieties, thereby influencing critical oncogenic processes such as DNA repair, stemness, and apoptosis resistance. This compound specifically inhibits UCHL3 activity, leading to the destabilization of substrates like the aryl hydrocarbon receptor (AhR) and suppression of cancer stem-like properties .
Preparation Methods
Temperature Cycle-Induced Deracemization (TCID) of Axially Chiral Naphthamide Derivatives
Principles of this compound Methodology
Temperature Cycle-Induced Deracemization leverages reversible solubility changes during heating-cooling cycles to amplify enantiomeric excess in crystalline phases. For the naphthamide derivative studied, the process involves:
- Initial Crystallization : A racemic suspension undergoes temperature fluctuations between 30°C and 60°C, inducing partial dissolution and recrystallization.
- Chirality Amplification : Over 15–20 cycles, the crystalline phase achieves >99% enantiomeric excess (ee) due to preferential growth of one enantiomer’s crystals.
- Semi-Continuous Operation : After deracemization, 80% of the suspension is harvested, and fresh racemic feedstock is added to the remaining 20% seed crystals for subsequent batches.
Key Process Parameters:
This method achieved a productivity of 7.71 g·L⁻¹·h⁻¹, demonstrating scalability for batch crystallizers.
Synthesis of Anti-Norovirus Thienyl Benzothiazolyl Carboxamides
Reaction Scheme and Optimization
The synthesis involves coupling substituted thiophene carboxylic acids with fluorinated benzothiazol-2-amines (Figure 1):
Figure 1: General synthesis route for carboxamide derivatives
Thiophene-2-carboxylic acid + 6-Fluorobenzo[d]thiazol-2-amine
→ EDC·HCl, DMAP, CH₂Cl₂
→ Target carboxamide (e.g., Compound 2b)
Critical Synthetic Steps:
- Coupling Agent Selection : Ethylcarbodiimide hydrochloride (EDC·HCl) with 4-dimethylaminopyridine (DMAP) gave superior yields (40–43%) compared to other activating agents.
- Halogenation Effects :
Representative Compound Data
Table 1: Biological activity of selected carboxamides
Compound | Thiophene Substituent | Benzothiazole Substituent | EC₅₀ (µM) | CC₅₀ (µM) |
---|---|---|---|---|
2b | 5-Cl | 6-F | 30 | >100 |
2k | 3,5-Cl | 6-F | 6.6 | >100 |
4b | 5-Br, 3,5-Cl | 4,6-F | 0.53 | 89 |
Analytical and Evaluation Methodologies
TCID50 Assay for Antiviral Activity
The Tissue Culture Infectious Dose 50% (TCID50) protocol quantifies compound efficacy through:
- Viral Titration : Serial 10-fold dilutions of norovirus stocks in TZM-bl cells.
- Endpoint Determination : Luminometric detection after 48h incubation using Britelite™ substrate.
- Calculations : Reed-Muench method determines the dilution reducing infectivity by 50% compared to controls.
For compound 4b , pretreatment with 1 µM reduced viral titers by 2.5 log₁₀ TCID50/mL, confirming direct virucidal effects.
Comparative Analysis of Deracemization vs. Directed Synthesis
Table 2: Process characteristics comparison
Industrial Application Considerations
Deracemization Process Economics
- Batch Utilization : Existing crystallizers require minimal modification for semi-continuous this compound operation.
- Solvent Consumption : Ethanol/water mixtures reduce costs vs. chiral chromatography.
Carboxamide Production Challenges
Chemical Reactions Analysis
Types of Reactions: 4,5,6,7-Tetrachloroindan-1,3-dione undergoes various chemical reactions, including:
Oxidation: The compound can be oxidized to form higher oxidation state derivatives.
Reduction: Reduction reactions can lead to the formation of less chlorinated indan-1,3-dione derivatives.
Substitution: Halogen substitution reactions can occur, where chlorine atoms are replaced by other functional groups.
Common Reagents and Conditions:
Oxidation: Common oxidizing agents include potassium permanganate and chromium trioxide.
Reduction: Reducing agents such as lithium aluminum hydride and sodium borohydride are used.
Substitution: Nucleophilic substitution reactions often involve reagents like sodium methoxide or potassium tert-butoxide.
Major Products: The major products formed from these reactions depend on the specific reagents and conditions used. For example, oxidation may yield tetrachloroindan-1,3-dione derivatives with additional oxygen-containing functional groups, while reduction may produce partially dechlorinated indan-1,3-dione compounds.
Scientific Research Applications
Virology and Infectious Disease Research
TCID is primarily utilized in virology to assess viral infectivity. The TCID50 assay measures the dilution at which 50% of the cells exhibit cytopathic effects (CPE). This method is essential for:
- Virus Characterization : Determining the virulence and pathogenicity of different viral strains.
- Vaccine Development : Evaluating the efficacy of vaccines by measuring the reduction in TCID50 after vaccination.
- Antiviral Drug Testing : Assessing the effectiveness of antiviral compounds by comparing TCID50 values before and after treatment with potential drugs.
For instance, a study highlighted the use of TCID50 to establish antiviral activity against Respiratory Syncytial Virus (RSV), demonstrating consistent results over extended periods, which is critical for high-throughput screening (HTS) campaigns .
Drug Development and Screening
In pharmaceutical research, this compound plays a pivotal role in drug discovery and development:
- High-Throughput Screening : this compound assays can be adapted for HTS to evaluate large libraries of compounds for antiviral activity. For example, using focused infectious clones (FICs) instead of live viruses has been shown to enhance assay robustness against unstable viruses .
- Case Studies : Research on Porcine Reproductive and Respiratory Syndrome Virus (PRRSV) employed TCID50 to identify effective anti-viral compounds, showcasing its utility in screening potential therapeutic agents .
Industrial Applications
Beyond laboratory settings, this compound methodologies have implications in industrial processes:
- Food Safety : The stability of glycosidic bonds in food matrices has been studied using this compound techniques to assess the safety and stability of food additives like steviol glycosides. This ensures that food products maintain their integrity during processing .
- Biotechnology : Recent advancements have demonstrated that temperature-cycle-induced deracemization (this compound) can enhance yields in industrial chemical processes. This application focuses on optimizing conditions for asymmetric synthesis, crucial for producing chiral compounds used in agrochemicals .
Emerging Technologies
Recent innovations have integrated this compound with advanced imaging techniques, such as Total Internal Reflection Fluorescence (TIRF) microscopy combined with Lab-on-a-Chip technology. This integration allows for real-time observation of viral interactions at the single-molecule level, significantly enhancing our understanding of viral dynamics and host interactions .
Data Table: Summary of this compound Applications
Application Area | Description | Key Findings/Case Studies |
---|---|---|
Virology | Measures viral infectivity via TCID50 | Essential for vaccine efficacy and virus characterization |
Drug Development | Used in HTS to identify antiviral compounds | Successful screening against RSV using FICs |
Food Safety | Assesses stability of food additives | Stability studies on steviol glycosides in various matrices |
Industrial Biotechnology | Enhances yields in chemical synthesis through temperature cycling | Improved deracemization processes for chiral compound production |
Mechanism of Action
The primary mechanism of action of 4,5,6,7-Tetrachloroindan-1,3-dione involves the inhibition of UCH-L3, an enzyme that plays a crucial role in the ubiquitin-proteasome system. By inhibiting UCH-L3, TCID reduces the ubiquitination of glycine transporter GlyT2 in neurons, which can affect neurotransmitter regulation and neuronal function .
Comparison with Similar Compounds
TCID belongs to the emerging class of deubiquitinase (DUB) inhibitors. Below is a comparative analysis with other DUB-targeting compounds, focusing on specificity, mechanisms, and therapeutic outcomes:
Table 1: Comparison of this compound with Other DUB Inhibitors
Key Differentiators of this compound:
- Specificity : Unlike pan-DUB inhibitors (e.g., PR-619), this compound selectively targets UCHL3, minimizing off-target effects .
Research Findings and Data Tables
Table 2: Preclinical Efficacy of this compound in Cancer Models
Table 3: this compound’s Impact on Biomarkers
Biological Activity
TCID, or Temperature Cycle Induced Deracemization , primarily functions as a selective inhibitor of ubiquitin C-terminal hydrolase-L3 (UCH-L3). This compound has garnered attention in the field of biochemistry for its specific biological activities and potential applications in therapeutic contexts.
This compound exhibits a potent inhibitory effect on UCH-L3, with an IC50 value of 0.6 μM , indicating its effectiveness at low concentrations. The compound shows over 100-fold selectivity for UCH-L3 compared to UCH-L1, which is significant for targeted therapeutic strategies aimed at modulating protein degradation pathways in various diseases, including neurodegenerative disorders and cancers .
Biological Implications
The inhibition of UCH-L3 by this compound has been linked to the modulation of glycine transporter GlyT2 ubiquitination in primary neurons from the brain stem and spinal cord. This process is crucial for maintaining neurotransmitter homeostasis and neuronal function. Research indicates that this compound diminishes the ubiquitination of GlyT2, potentially influencing synaptic transmission and plasticity .
Case Studies and Research Findings
Several studies have explored the biological activity of this compound in different contexts:
- Neuronal Function : A study by de Juan-Sanz et al. (2013) demonstrated that the turnover of GlyT2 is dependent on its ubiquitination status, which can be modulated by this compound. The inhibition of UCH-L3 by this compound may thus provide insights into therapeutic approaches for disorders characterized by altered glycine signaling .
- Antiviral Activity : Although not directly related to this compound as a compound, the concept of TCID50 (50% tissue culture infectious dose) has been widely used in virology to measure viral infectivity. For instance, research involving UV light treatment has utilized TCID50 assays to assess the efficacy of viral inactivation methods, highlighting the importance of understanding viral dynamics in relation to compounds like this compound .
Data Summary
The following table summarizes key findings related to the biological activity of this compound:
Parameter | Value |
---|---|
Target Enzyme | UCH-L3 |
IC50 | 0.6 μM |
Selectivity Ratio | >100-fold over UCH-L1 |
Biological Role | Modulates GlyT2 ubiquitination |
Potential Applications | Neurodegenerative diseases, cancer therapy |
Q & A
Basic Research Questions
Q. What are the critical experimental parameters to optimize TCID for enantiomer separation?
this compound requires precise control of temperature cycles, initial enantiomeric excess (c.e.e0), solute concentration, and cooling rates. For example, c.e.e0 values ≥2% reduce induction times and ensure directional deracemization, while slower cooling rates prevent unintended nucleation. Analytical methods like chiral HPLC or circular dichroism are essential for real-time monitoring of enantiopurity .
Q. How should researchers design a this compound experiment to validate theoretical yield claims?
Q. What analytical techniques are recommended for assessing enantiomeric purity during this compound?
Chiral high-performance liquid chromatography (HPLC) is the gold standard for quantifying c.e.e. during this compound. Researchers must calibrate instruments with racemic and pure enantiomer standards. Spectroscopic methods like circular dichroism or polarimetry can supplement HPLC but require validation against chromatographic data .
Advanced Research Questions
Q. How can chiral flipping risks be mitigated in this compound experiments with low c.e.e0?
Chiral flipping occurs when c.e.e0 ≤1%, as stochastic nucleation biases deracemization direction. To mitigate this, maintain c.e.e0 ≥2% and apply controlled cooling rates (e.g., ≤0.5°C/min) to suppress primary nucleation. shows that c.e.e0 = +1.4% led to flipping, while c.e.e0 ≥2% ensured stable deracemization .
Q. What methodologies resolve contradictions between this compound’s theoretical and practical yields?
Discrepancies arise from solute decomposition, incomplete crystal washing, or scale-dependent cooling inefficiencies. Researchers should:
- Conduct thermogravimetric analysis to rule out decomposition.
- Optimize crystal washing protocols to recover dissolved solute (e.g., achieved 92% yield with post-TCID cooling).
- Validate scalability by comparing lab (2.5g) and pilot-scale (8.75g) results under identical conditions .
Q. How can induction time variability in this compound be systematically addressed?
Induction time depends on c.e.e0 and crystal size distribution. To minimize variability:
- Pre-seed experiments with enantiopure crystals to bypass nucleation delays.
- Use computational models (e.g., Bodák et al.’s size-distribution analysis) to predict deracemization trajectories.
- found no induction time for c.e.e0 ≥1%, enabling faster deracemization .
Q. What statistical frameworks are suitable for analyzing this compound data variability?
Reed-Muench or Spearman-Karber methods (traditionally used in virology this compound50 assays) can be adapted to calculate effective deracemization thresholds. For reproducibility, report 95% confidence intervals and use ANOVA to compare yield variances across experimental replicates .
Q. Methodological Best Practices
- Data Presentation : Follow journal guidelines (e.g., Journal of Chemical Sciences) for structuring tables, including raw data, c.e.e. calculations, and error margins. Embed figures showing temperature cycles and yield trends .
- Ethical Reporting : Disclose conflicts of interest and ensure reproducibility by detailing solvent purity, equipment specifications, and crystal-washing protocols .
- Literature Review : Use ORCID-linked databases (e.g., Web of Science) to track this compound advancements and avoid redundant hypotheses .
Properties
IUPAC Name |
4,5,6,7-tetrachloroindene-1,3-dione | |
---|---|---|
Source | PubChem | |
URL | https://pubchem.ncbi.nlm.nih.gov | |
Description | Data deposited in or computed by PubChem | |
InChI |
InChI=1S/C9H2Cl4O2/c10-6-4-2(14)1-3(15)5(4)7(11)9(13)8(6)12/h1H2 | |
Source | PubChem | |
URL | https://pubchem.ncbi.nlm.nih.gov | |
Description | Data deposited in or computed by PubChem | |
InChI Key |
IDLAOWFFKWRNHB-UHFFFAOYSA-N | |
Source | PubChem | |
URL | https://pubchem.ncbi.nlm.nih.gov | |
Description | Data deposited in or computed by PubChem | |
Canonical SMILES |
C1C(=O)C2=C(C1=O)C(=C(C(=C2Cl)Cl)Cl)Cl | |
Source | PubChem | |
URL | https://pubchem.ncbi.nlm.nih.gov | |
Description | Data deposited in or computed by PubChem | |
Molecular Formula |
C9H2Cl4O2 | |
Source | PubChem | |
URL | https://pubchem.ncbi.nlm.nih.gov | |
Description | Data deposited in or computed by PubChem | |
DSSTOX Substance ID |
DTXSID80369561 | |
Record name | 4,5,6,7-Tetrachloro-1H-indene-1,3(2H)-dione | |
Source | EPA DSSTox | |
URL | https://comptox.epa.gov/dashboard/DTXSID80369561 | |
Description | DSSTox provides a high quality public chemistry resource for supporting improved predictive toxicology. | |
Molecular Weight |
283.9 g/mol | |
Source | PubChem | |
URL | https://pubchem.ncbi.nlm.nih.gov | |
Description | Data deposited in or computed by PubChem | |
CAS No. |
30675-13-9 | |
Record name | 4,5,6,7-Tetrachloro-1H-indene-1,3(2H)-dione | |
Source | EPA DSSTox | |
URL | https://comptox.epa.gov/dashboard/DTXSID80369561 | |
Description | DSSTox provides a high quality public chemistry resource for supporting improved predictive toxicology. | |
Synthesis routes and methods
Procedure details
Retrosynthesis Analysis
AI-Powered Synthesis Planning: Our tool employs the Template_relevance Pistachio, Template_relevance Bkms_metabolic, Template_relevance Pistachio_ringbreaker, Template_relevance Reaxys, Template_relevance Reaxys_biocatalysis model, leveraging a vast database of chemical reactions to predict feasible synthetic routes.
One-Step Synthesis Focus: Specifically designed for one-step synthesis, it provides concise and direct routes for your target compounds, streamlining the synthesis process.
Accurate Predictions: Utilizing the extensive PISTACHIO, BKMS_METABOLIC, PISTACHIO_RINGBREAKER, REAXYS, REAXYS_BIOCATALYSIS database, our tool offers high-accuracy predictions, reflecting the latest in chemical research and data.
Strategy Settings
Precursor scoring | Relevance Heuristic |
---|---|
Min. plausibility | 0.01 |
Model | Template_relevance |
Template Set | Pistachio/Bkms_metabolic/Pistachio_ringbreaker/Reaxys/Reaxys_biocatalysis |
Top-N result to add to graph | 6 |
Feasible Synthetic Routes
Disclaimer and Information on In-Vitro Research Products
Please be aware that all articles and product information presented on BenchChem are intended solely for informational purposes. The products available for purchase on BenchChem are specifically designed for in-vitro studies, which are conducted outside of living organisms. In-vitro studies, derived from the Latin term "in glass," involve experiments performed in controlled laboratory settings using cells or tissues. It is important to note that these products are not categorized as medicines or drugs, and they have not received approval from the FDA for the prevention, treatment, or cure of any medical condition, ailment, or disease. We must emphasize that any form of bodily introduction of these products into humans or animals is strictly prohibited by law. It is essential to adhere to these guidelines to ensure compliance with legal and ethical standards in research and experimentation.