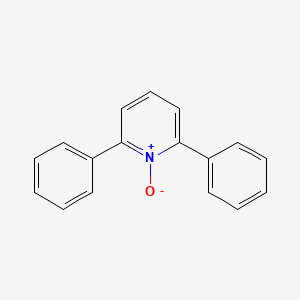
2,6-Diphenylpyridine N-oxide
Overview
Description
2,6-Diphenylpyridine N-oxide is a heterocyclic compound characterized by a pyridine core functionalized with phenyl groups at the 2- and 6-positions and an N-oxide moiety. Its structure imparts unique electronic properties, such as enhanced polarity and electron-deficient characteristics, making it valuable in diverse applications. The phenyl substituents confer steric bulk and modulate electron density, distinguishing it from simpler pyridine N-oxide derivatives.
Preparation Methods
Synthetic Routes and Reaction Conditions
The synthesis of 2,6-Diphenylpyridine N-oxide typically involves the oxidation of 2,6-diphenylpyridine. One common method uses sodium percarbonate as an oxygen source in the presence of rhenium-based catalysts under mild reaction conditions . Another method employs titanium silicalite (TS-1) in a packed-bed microreactor with hydrogen peroxide in methanol as the solvent, offering a safer and more efficient process .
Industrial Production Methods
Industrial production methods for this compound often focus on optimizing yield and safety. The use of solvent-free protocols and reusable heterogeneous catalysts, such as activated Fuller’s earth, has been explored to enhance efficiency and reduce environmental impact .
Chemical Reactions Analysis
Nucleophilic Oxidation Reactions
2,6-Diphenylpyridine N-oxide acts as a potent oxidant in metal-catalyzed systems. For example, in gold(I)-catalyzed reactions, it facilitates the oxidation of alkynes to form α-oxo gold carbenes. This mechanism involves:
-
Step 1 : Nucleophilic attack on the alkyne by the N-oxide, forming a covalent intermediate.
-
Step 2 : Fragmentation of the N–O bond, generating an electrophilic α-oxo gold carbene.
-
Step 3 : Trapping by nucleophiles (e.g., hydroxy groups) to yield oxidized products .
Reaction Type | Catalyst | Key Reaction Feature | Yield (Example) | Reference |
---|---|---|---|---|
Alkyne Oxidation | Au(I) | Formation of α-oxo gold carbene intermediate | Not specified |
Deoxygenation Methods
The N–O bond in this compound can be cleaved using reductive agents:
-
Phosphorus oxychloride (POCl₃) : Converts the N-oxide to pyridine derivatives under alkaline conditions .
-
Trifluoroacetic acid anhydride : Reduces the N-oxide to pyridine via nucleophilic displacement .
-
Aluminum iodide (AlI₃) : Acts as an economic reagent for N–O bond cleavage .
Reagent | Reaction Conditions | Product | Reference |
---|---|---|---|
POCl₃/K₂CO₃ | Reflux in CHCl₃ | Pyridine derivatives | |
(CF₃CO)₂O/NaI | CH₃CN, 0°C | Pyridine |
Electrophilic Substitution and Nitration
The aromatic ring of this compound undergoes electrophilic substitution due to the activating effect of the N-oxide group. For example:
-
Nitration : Using HNO₃/H₂SO₄, the N-oxide directs electrophilic attack to the para position relative to the N-oxide group .
-
Substitution patterns : Steric hindrance from the phenyl groups at positions 2 and 6 influences reactivity.
Reaction Type | Reagent | Position of Substitution | Product | Reference |
---|---|---|---|---|
Nitration | HNO₃/H₂SO₄ | Para to N-oxide | 4-Nitro derivative |
Cycloaddition Reactions
Pyridine N-oxides participate in cycloadditions due to their electron-deficient aromatic rings. For example:
-
Diels-Alder reactions : The N-oxide acts as a dienophile, forming six-membered rings .
-
1,3-Dipolar cycloadditions : Potential reactivity with dipolar species under thermal or photochemical conditions .
Reactivity and Stability Insights
Computational studies reveal:
-
Bond dissociation energy (BDE) : The N–O bond in pyridine N-oxides has a BDE of ~60–66 kcal/mol, influenced by conjugation and resonance .
-
Proton affinity : Pyridine N-oxides exhibit lower proton affinities compared to aliphatic amine oxides, affecting their acidity in biological media .
Property | Value (kcal/mol) | Comparison to Aliphatic N-Oxides | Reference |
---|---|---|---|
N–O BDE | ~60–66 | Higher than trimethylamine N-oxide | |
Proton Affinity | Lower | Due to resonance stabilization |
Scientific Research Applications
Synthesis and Catalysis
1.1 C-H Arylation Reactions
DPPO has been utilized as a coupling partner in copper-catalyzed direct C-H arylation reactions. This method allows for the synthesis of 2-arylpyridines from arylboronic esters with moderate to good yields. The reaction is notable for its efficiency, requiring no additional reductants, thus simplifying the synthetic process .
Table 1: Summary of C-H Arylation Yields
Arylboronic Ester | Yield (%) |
---|---|
Para-tolylboronic | 84 |
Meta-tolylboronic | 89 |
Ortho-tolylboronic | 41 |
This table illustrates the impact of substituent position on yield, indicating that para- and meta-substituted arylboronic esters generally provide higher yields compared to ortho-substituted ones.
Coordination Chemistry
2.1 Ligand Properties
DPPO acts as a tridentate ligand in coordination complexes, particularly with transition metals like gold(III). Its ability to stabilize metal centers through multiple coordination sites enhances the reactivity and selectivity of the resulting complexes .
Case Study: Gold(III) Complexes
A series of cyclometalated gold(III) complexes were synthesized using DPPO as a ligand. These complexes exhibited unique structural and spectroscopic properties, demonstrating the effectiveness of DPPO in stabilizing metal centers and facilitating catalytic processes .
Photopolymerization Applications
3.1 Cationic Photopolymerization
DPPO derivatives have shown promise in accelerating cationic photopolymerization processes. These compounds can enhance the polymerization rate when used as photoinitiators, making them valuable in the development of advanced materials .
Table 2: Performance of DPPO Derivatives in Photopolymerization
Compound | Polymerization Rate (cm/s) | Sensitivity (Fluorescence Ratio) |
---|---|---|
DPPO Derivative S1 | 0.15 | 2.5 |
DPPO Derivative S2 | 0.20 | 3.0 |
The above data indicates that variations in substituents on DPPO derivatives significantly affect both the polymerization rate and sensitivity, highlighting their potential in real-time monitoring applications.
Biological Applications
4.1 Bioactive Compound Development
Pyridine N-oxides, including DPPO, have been explored for their bioactive properties. They serve as precursors for developing various pharmacologically active compounds due to their ability to undergo selective functionalizations .
Case Study: Synthesis of Bioactive Pyridine Derivatives
Research has shown that utilizing DPPO in reactions can yield bioactive compounds with high regioselectivity and good yields, making them suitable candidates for drug development .
Mechanism of Action
The mechanism of action of 2,6-Diphenylpyridine N-oxide involves its role as a mild Lewis base. It can activate certain Lewis acidic parts of molecules, increasing the reactivity of nucleophilic parts towards electrophiles . This activation facilitates various chemical reactions, including oxidation and substitution.
Comparison with Similar Compounds
Substituent Effects on Fluorescence Properties
Electron-donating and withdrawing substituents significantly influence fluorescence behavior in pyridine N-oxide derivatives. For instance:
- 2,6-Diphenylpyridine N-oxide derivatives with -SCH₃ groups (e.g., PT-SCH₃) exhibit strong positive solvatochromism and enable real-time monitoring of free-radical polymerization via fluorescence intensity shifts. Their electron-donating substituents enhance sensitivity to polymerization progress .
- Electron-withdrawing substituents (e.g., -Cl, -NO₂) in analogs like 2,6-dimethyl-4-nitropyridine N-oxide result in minimal fluorescence changes during polymerization, limiting their utility as sensors .
In contrast, 2,6-dichloropyridine N-oxide (non-fluorescent) is primarily employed in catalysis rather than sensing, underscoring how substituent choice dictates application .
Spectral Characteristics and Solvent Interactions
UV-spectral studies reveal substituent-dependent protonation equilibria in acetonitrile:
- 2,6-Dimethyl-4-methoxypyridine N-oxide displays distinct absorption bands due to equilibrium between protonated N-oxide and free base forms, influenced by electron-donating methoxy groups .
Data Tables
Table 1: Substituent Effects on Key Properties of Pyridine N-Oxide Derivatives
Table 2: Spectral and Physical Properties
Biological Activity
2,6-Diphenylpyridine N-oxide (2,6-DPN) is a compound that has garnered attention in various scientific fields due to its unique structural properties and biological activities. This article explores the biological activity of 2,6-DPN, including its mechanism of action, applications in research and medicine, and relevant case studies.
Chemical Structure and Properties
The molecular formula of 2,6-DPN is . Its structure features a pyridine ring with two phenyl groups at the 2 and 6 positions, along with an N-oxide functional group. This configuration contributes to its reactivity and interactions with biological molecules.
Biochemical Pathways
2,6-DPN acts as a fluorescent chemosensor , particularly for detecting silver ions (Ag+) in aqueous solutions. Its high selectivity and significant fluorescent response make it valuable for monitoring Ag+ in mammalian cells. The compound's ability to shift its fluorescence spectrum during free-radical polymerization allows for tracking polymerization processes through fluorescence intensity measurements.
Interaction with Biological Molecules
The unique structure of 2,6-DPN enables it to interact with various biological molecules, facilitating studies on enzyme mechanisms and metabolic pathways. Its role as a mild oxidant in organic synthesis further underscores its versatility in biochemical applications.
Applications in Research and Medicine
2,6-DPN has been utilized in several scientific domains:
- Chemistry : As a reagent in organic synthesis, particularly in oxidation reactions.
- Biology : Useful for studying enzyme mechanisms and metabolic pathways.
- Medicine : Investigated for potential therapeutic applications due to its ability to modulate biological processes.
- Industry : Employed in developing fluorescent sensors for monitoring photopolymerization processes.
Antileishmanial Activity
A study evaluated the antileishmanial activity of various N-oxide derivatives, including those related to 2,6-DPN. The results indicated that certain derivatives exhibited significant efficacy against Leishmania infantum, with one compound showing an EC50 value of 3.6 μM against amastigote forms. In vivo studies demonstrated a reduction of approximately 90% in parasite burden in infected mice .
Antitubercular Potential
Research involving N-oxide-containing compounds highlighted their potential against Mycobacterium tuberculosis. One derivative demonstrated promising results with MIC90 values of 1.10 μM against active Mtb strains. In vivo experiments confirmed its effectiveness in reducing bacterial loads significantly .
Antiproliferative Activity
A series of studies focused on the synthesis and evaluation of imidazo[4,5-b]pyridines derived from 2,6-DPN. These derivatives exhibited strong antiproliferative activity against various cancer cell lines. For instance, one derivative showed an IC50 range of 1.45–4.25 μM across multiple tested cell lines, indicating potential for further development as anticancer agents .
Summary of Biological Activities
Q & A
Q. Basic: What synthetic methodologies are employed to prepare 2,6-diphenylpyridine N-oxide, and how is purity validated?
Answer:
this compound is typically synthesized via oxidation of 2,6-diphenylpyridine using meta-chloroperbenzoic acid (mCPBA) as the oxidizing agent . Purification involves column chromatography or recrystallization. Purity is confirmed using high-performance liquid chromatography (HPLC) and nuclear magnetic resonance (NMR) spectroscopy (¹H/¹³C). Mass spectrometry (MS) further validates molecular integrity by confirming the molecular ion peak (M⁺) and fragmentation pattern .
Q. Basic: Which spectroscopic techniques are critical for characterizing this compound’s structure and electronic properties?
Answer:
Key techniques include:
- UV-Vis spectroscopy : Identifies π→π* and n→π* transitions, with solvatochromic shifts observed in polar solvents .
- Fluorescence spectroscopy : Evaluates emission properties, particularly for derivatives with electron-donating/withdrawing groups .
- NMR : ¹H and ¹³C NMR in deuterated solvents (e.g., CDCl₃, DMSO-d₆) confirm substituent effects on aromatic protons and carbon environments .
- X-ray crystallography : Resolves solid-state geometry, particularly when coordinated to metal centers .
Q. Basic: How is the lipophilicity (logP) of this compound experimentally determined?
Answer:
The 1-octanol/water partition coefficient (logP) is measured via shake-flask method or reverse-phase HPLC using calibrated standards. Retention times correlate with hydrophobicity, as seen in analogous pyridine derivatives (e.g., logP = 4.9 for 2,6-diphenylpyridine) . For N-oxide derivatives, polar N-O bonds reduce logP compared to parent pyridines.
Q. Basic: What role does this compound play in coordination chemistry?
Answer:
It acts as a tridentate [C^N^C] dianionic ligand , forming stable cyclometalated complexes with transition metals (e.g., Pt(II), Au(III), Os(IV)). The ligand’s rigidity enhances photophysical properties in metal complexes, such as luminescence and catalytic activity. Structural characterization via X-ray diffraction reveals bond lengths and bite angles critical for tuning reactivity .
Q. Advanced: How do substituents on the pyridine ring influence the reactivity of metal complexes?
Answer:
Modifications like oxygen insertion (e.g., 2-phenoxy-6-phenylpyridine) alter ligand bite angles and electronic properties. For example:
- Iridium(III) complexes with phenoxy substituents show enhanced catalytic efficiency in C-H activation .
- Osmium(IV) complexes exhibit reduced reactivity due to steric and electronic effects .
Kinetic studies (e.g., time-resolved UV-Vis) quantify substituent impacts on reaction rates .
Q. Advanced: What mechanistic insights exist for this compound in catalytic applications?
Answer:
In oxidation reactions, the N-oxide group participates in oxygen transfer via high-valent metal-oxo intermediates. For example:
- Ru-porphyrin systems leverage N-oxide ligands for selective alkene epoxidation, with turnover numbers (TON) monitored via GC-MS .
- Mechanistic probes (e.g., isotopic labeling, EPR spectroscopy) identify radical intermediates in mCPBA-mediated oxidations .
Q. Advanced: How is cytotoxicity evaluated for metal complexes derived from this compound?
Answer:
Cytotoxicity assays (e.g., MTT, SRB) are performed on cancer cell lines (e.g., HeLa, MCF-7) with IC₅₀ values calculated. For example:
- Platinum(II) complexes show dose-dependent toxicity, with apoptosis confirmed via flow cytometry .
- Structure-activity relationships (SAR) link ligand hydrophobicity to cellular uptake efficiency .
Q. Advanced: What methodologies assess the antimutagenic potential of pyridine N-oxide derivatives?
Answer:
The Ames test (fluctuation method) evaluates mutagenicity using Salmonella typhimurium strains (e.g., TA98, TA100). Antigenotoxic effects are quantified by comparing mutation rates induced by mutagens (e.g., 2-AA, 4-NQO) with/without the N-oxide compound. Statistical analysis (e.g., ANOVA) validates significance .
Q. Advanced: How does solvatochromism inform the design of 2,6-diphenylpyridine-based sensors?
Answer:
Solvatochromic shifts in emission spectra (e.g., PT-SCH₃ derivatives) correlate with solvent polarity (ET(30) scale). Time-dependent density functional theory (TD-DFT) models predict excited-state behavior, guiding the design of ratiometric sensors for environmental pollutants .
Q. Advanced: How are synthetic routes optimized for high-yield this compound production?
Answer:
One-pot syntheses minimize intermediates, as demonstrated in condensation reactions between phenylacetaldehyde and N-chlorophenylacetaldimine . Reaction parameters (pH, temperature, catalyst loading) are optimized via Design of Experiments (DoE), with yields monitored by HPLC .
Properties
IUPAC Name |
1-oxido-2,6-diphenylpyridin-1-ium | |
---|---|---|
Source | PubChem | |
URL | https://pubchem.ncbi.nlm.nih.gov | |
Description | Data deposited in or computed by PubChem | |
InChI |
InChI=1S/C17H13NO/c19-18-16(14-8-3-1-4-9-14)12-7-13-17(18)15-10-5-2-6-11-15/h1-13H | |
Source | PubChem | |
URL | https://pubchem.ncbi.nlm.nih.gov | |
Description | Data deposited in or computed by PubChem | |
InChI Key |
FBLQGULBXCFSAK-UHFFFAOYSA-N | |
Source | PubChem | |
URL | https://pubchem.ncbi.nlm.nih.gov | |
Description | Data deposited in or computed by PubChem | |
Canonical SMILES |
C1=CC=C(C=C1)C2=[N+](C(=CC=C2)C3=CC=CC=C3)[O-] | |
Source | PubChem | |
URL | https://pubchem.ncbi.nlm.nih.gov | |
Description | Data deposited in or computed by PubChem | |
Molecular Formula |
C17H13NO | |
Source | PubChem | |
URL | https://pubchem.ncbi.nlm.nih.gov | |
Description | Data deposited in or computed by PubChem | |
DSSTOX Substance ID |
DTXSID70508149 | |
Record name | 1-Oxo-2,6-diphenyl-1lambda~5~-pyridine | |
Source | EPA DSSTox | |
URL | https://comptox.epa.gov/dashboard/DTXSID70508149 | |
Description | DSSTox provides a high quality public chemistry resource for supporting improved predictive toxicology. | |
Molecular Weight |
247.29 g/mol | |
Source | PubChem | |
URL | https://pubchem.ncbi.nlm.nih.gov | |
Description | Data deposited in or computed by PubChem | |
CAS No. |
78500-88-6 | |
Record name | 1-Oxo-2,6-diphenyl-1lambda~5~-pyridine | |
Source | EPA DSSTox | |
URL | https://comptox.epa.gov/dashboard/DTXSID70508149 | |
Description | DSSTox provides a high quality public chemistry resource for supporting improved predictive toxicology. | |
Synthesis routes and methods
Procedure details
Retrosynthesis Analysis
AI-Powered Synthesis Planning: Our tool employs the Template_relevance Pistachio, Template_relevance Bkms_metabolic, Template_relevance Pistachio_ringbreaker, Template_relevance Reaxys, Template_relevance Reaxys_biocatalysis model, leveraging a vast database of chemical reactions to predict feasible synthetic routes.
One-Step Synthesis Focus: Specifically designed for one-step synthesis, it provides concise and direct routes for your target compounds, streamlining the synthesis process.
Accurate Predictions: Utilizing the extensive PISTACHIO, BKMS_METABOLIC, PISTACHIO_RINGBREAKER, REAXYS, REAXYS_BIOCATALYSIS database, our tool offers high-accuracy predictions, reflecting the latest in chemical research and data.
Strategy Settings
Precursor scoring | Relevance Heuristic |
---|---|
Min. plausibility | 0.01 |
Model | Template_relevance |
Template Set | Pistachio/Bkms_metabolic/Pistachio_ringbreaker/Reaxys/Reaxys_biocatalysis |
Top-N result to add to graph | 6 |
Feasible Synthetic Routes
Disclaimer and Information on In-Vitro Research Products
Please be aware that all articles and product information presented on BenchChem are intended solely for informational purposes. The products available for purchase on BenchChem are specifically designed for in-vitro studies, which are conducted outside of living organisms. In-vitro studies, derived from the Latin term "in glass," involve experiments performed in controlled laboratory settings using cells or tissues. It is important to note that these products are not categorized as medicines or drugs, and they have not received approval from the FDA for the prevention, treatment, or cure of any medical condition, ailment, or disease. We must emphasize that any form of bodily introduction of these products into humans or animals is strictly prohibited by law. It is essential to adhere to these guidelines to ensure compliance with legal and ethical standards in research and experimentation.