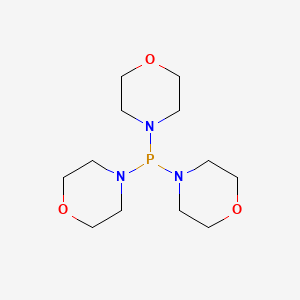
Tris(morpholino)phosphine
Overview
Description
Tris(morpholino)phosphine (TMP) is a tertiary phosphine featuring three morpholine substituents bonded to a central phosphorus atom. Its synthesis often involves reactions with phosphorus trichloride and morpholine derivatives, as demonstrated in the reduction of phosphine selenides to phosphonous diamides (e.g., synthesis of compounds 6a and 6b via TMP in benzene) . Structurally, TMP exhibits a trigonal pyramidal geometry, with P–N bond lengths averaging ~1.70–1.71 Å in its selenide and telluride forms, as determined by X-ray crystallography .
TMP is notable for its dual role as a reducing agent in organic synthesis and a ligand in catalysis. Additionally, TMP derivatives are employed in ruthenium olefin metathesis catalysts, where ligand dissociation rates influence catalytic efficiency .
Preparation Methods
General Synthetic Approaches to Tris(morpholino)phosphine
The synthesis of this compound generally follows nucleophilic substitution reactions on phosphorus halides, such as phosphorus trichloride (PCl3), with morpholine acting as the nucleophile. This approach is consistent with the broader class of aminophosphines synthesis, where amines substitute halides on phosphorus centers under controlled conditions.
Stepwise substitution of PCl3 with morpholine: Morpholine is added dropwise to a cooled solution of PCl3, often in an inert solvent like hexane or dichloromethane, under an inert atmosphere (e.g., nitrogen) to avoid oxidation or hydrolysis. The reaction temperature is typically maintained low (0°C to room temperature) to control reaction rates and minimize side reactions.
Use of bases to scavenge HCl: During the substitution, hydrogen chloride (HCl) is generated as a by-product. Bases such as triethylamine or sodium hydroxide are employed to neutralize HCl, improving yield and purity.
Isolation of this compound: After completion, the reaction mixture is worked up by filtration to remove ammonium salts, followed by solvent evaporation and purification steps such as recrystallization or distillation.
Detailed Preparation Procedure from Literature
A representative procedure adapted from peer-reviewed sources involves the following steps:
Starting Materials: Phosphorus trichloride (PCl3) and morpholine.
-
- Solvent: Typically hexane or dichloromethane.
- Temperature: Initially 0°C during addition, then room temperature for completion.
- Atmosphere: Inert gas (nitrogen or argon) to prevent oxidation.
- Molar Ratios: Morpholine is used in slight excess (e.g., 3.1 equivalents) to ensure complete substitution.
-
- PCl3 is dissolved in the solvent and cooled.
- Morpholine solution is added dropwise over 1-2 hours with stirring.
- The mixture is stirred at room temperature for 12-24 hours.
-
- The reaction mixture is filtered to remove precipitated ammonium chloride.
- The filtrate is concentrated under reduced pressure.
- The crude product is purified by vacuum distillation or recrystallization.
Yield: Typically ranges from 70% to 90%, depending on conditions and scale.
Reaction Parameters and Optimization
Parameter | Typical Range/Condition | Notes |
---|---|---|
Temperature | 0°C (addition) to 25°C (stirring) | Low temperature controls side reactions |
Solvent | Hexane, Dichloromethane | Non-polar solvents preferred |
Morpholine equivalents | 3.0 - 3.5 | Slight excess to drive substitution |
Reaction time | 12 - 24 hours | Ensures complete substitution |
Atmosphere | Nitrogen or Argon | Prevents oxidation of phosphine |
Base | Triethylamine or NaOH (optional) | Neutralizes HCl by-product |
Alternative Synthetic Routes
From Phosphine Oxides: this compound oxide can be synthesized by oxidation of this compound using mild oxidizing agents. However, the direct preparation of the phosphine is often preferred for ligand applications.
Using Quaternary Phosphonium Salts: Some patents describe the preparation of this compound derivatives via quaternary phosphonium salts, followed by hydrolysis under basic conditions (e.g., sodium hydroxide) to yield the free phosphine. This method allows fine control over substitution patterns but is more complex.
Characterization and Purity Assessment
-
- $$^{31}P$$-NMR chemical shifts for this compound typically appear near 20.5 ppm.
- $$^{1}H$$-NMR shows characteristic morpholine ring protons as triplets around 3.06 and 3.62 ppm.
- $$^{13}C$$-NMR confirms the morpholine carbons at approximately 67.3 ppm (O-CH2) and 45.2 ppm (N-CH2).
-
- Strong P–N and P–O related stretches are observed.
- For phosphine oxides, P=O stretch appears near 1031 cm$$^{-1}$$.
-
- The compound is often isolated as a colorless oil or crystalline solid depending on purity and preparation method.
Example Data Table: Synthesis and Characterization Summary
Compound | Yield (%) | $$^{31}P$$-NMR (ppm) | $$^{1}H$$-NMR (ppm) Morpholine CH2 | IR (P=O stretch, cm$$^{-1}$$) | Physical State |
---|---|---|---|---|---|
This compound | 85-90 | ~20.5 | 3.06 (t), 3.62 (t) | — | Colorless oil/crystal |
This compound oxide | 90+ | ~23.0 | 3.11 (t), 3.66 (t) | 1031 | Crystalline solid |
Research Findings on Preparation Efficiency and Stability
The use of inert atmosphere and controlled temperature is critical to prevent oxidation and degradation of the phosphine during synthesis.
Base selection for HCl scavenging affects the yield and purity; triethylamine is common, but sodium hydroxide can be used in hydrolysis steps for related phosphonium salts.
The reaction proceeds smoothly with high selectivity for trisubstitution without significant formation of mono- or disubstituted by-products under optimized conditions.
The prepared this compound serves as a hydrophilic ligand with potential applications in coordination chemistry, catalysis, and bioinorganic models due to its water solubility and electron-donating properties.
Chemical Reactions Analysis
Coordination Chemistry and Ligand Behavior
TMP demonstrates distinct coordination properties in metal complexes. In diiron propane-1,3-dithiolate complexes, TMP enhances hydrophilicity and protophilicity, making the complexes potential iron-only hydrogenase mimics . Key findings:
-
Electrochemical activity : TMP-ligated complexes exhibit electrocatalytic H₂ evolution in acetonitrile-water mixtures, with improved current sensitivity compared to non-aqueous solutions .
-
Ligand exchange : TMP participates in intermolecular ligand crossover reactions with other phosphine oxides (e.g., TOPO, TBP). The priority order for ligand replacement is TOPO > TBP > DEP > TMP .
Coordination Trends | Observations |
---|---|
Metal centers | Fe, Th(IV), La(III) |
Ligand strength (priority) | TOPO > TBP > DEP > TMP |
Stability in solution | TMP complexes decompose via multi-step elimination |
Stability and Decomposition Pathways
TMP derivatives undergo thermally induced decomposition, influenced by substituent electronic and steric effects:
-
Thermogravimetric analysis : TMP-ligated lanthanide/thorium complexes exhibit multi-step decomposition, releasing ligand fragments .
-
Hydrolysis : Free TMP undergoes partial hydrolysis at elevated temperatures, cleaving one morpholino substituent .
Stability Factors | Impact on Decomposition |
---|---|
Substituent size/electronics | Slower decomposition for bulkier groups |
Metal coordination | Stabilizes ligand in complexes |
Temperature | Accelerates cleavage of P–N bonds |
Ligand Crossover and Reactivity in Complexes
TMP exhibits competitive ligand exchange in mixed-metal systems:
-
Ligand transfer : TMP from La(III) complexes transfers to Th(IV) centers, forming mixed-ligand species .
-
Dynamic equilibrium : Reactions monitored via ³¹P-NMR reveal distinct signal shifts (e.g., δ 58.93 for Th(NO₃)₄(TOPO)(TBP)) .
Crossover Dynamics | Example System |
---|---|
Ligand priority | TOPO > TBP > DEP > TMP |
Metal center | Th(IV) vs. La(III) |
Reaction conditions | Heated at 70°C in chloroform |
Scientific Research Applications
Chemical Applications
1.1 Coordination Chemistry
TMP serves as a ligand in coordination chemistry, forming stable complexes with transition metals. These metal complexes exhibit unique electronic and steric properties that are beneficial in catalysis. For instance, TMP has been used to enhance the hydro- and protophilicity of iron-only hydrogenase active-site mimics, improving their electrocatalytic activity for proton reduction in mixed solvents like acetonitrile and water .
1.2 Catalysis
In catalytic applications, TMP has been investigated for its role in olefin metathesis reactions. Studies have shown that phosphine ligands like TMP can significantly influence the initiation rates of catalysts, thus affecting overall reaction efficiency . The incorporation of TMP into ruthenium-based catalysts has demonstrated improved activity due to its ability to stabilize metal centers and facilitate ligand dissociation during catalytic cycles .
Catalyst Type | Ligand | Activity Improvement |
---|---|---|
Ruthenium Olefin Metathesis | This compound | Enhanced initiation rates |
Iron Dithiolate Complexes | This compound | Improved electrocatalytic activity |
Biological Applications
2.1 Enzyme Mimics
TMP is employed in the development of enzyme mimics, particularly those that replicate the function of hydrogenases. Research has shown that TMP-modified diiron dithiolate complexes can effectively mimic the active sites of natural enzymes, showcasing significant electrocatalytic properties . This application holds promise for advancements in bio-inspired catalysis and renewable energy technologies.
2.2 Drug Delivery Systems
Ongoing research is exploring the potential of TMP in drug delivery systems due to its ability to form stable complexes with various therapeutic agents. The hydrophilic nature of TMP enhances its compatibility with biological systems, making it a candidate for developing targeted delivery mechanisms.
Industrial Applications
3.1 Organic Synthesis
In industrial settings, TMP is utilized as a reagent for synthesizing various organic compounds. Its ability to donate electrons through nitrogen atoms facilitates reactions that lead to the formation of phosphonium salts and other functionalized molecules. This characteristic is particularly useful in developing new materials and flame retardants.
3.2 Catalytic Processes
TMP's role as a catalyst in organic transformations is being actively researched. Its incorporation into catalytic systems has been shown to improve reaction selectivity and yield.
Case Study 1: Electrocatalytic Activity Enhancement
A study investigated the electrochemical properties of diiron complexes modified with TMP. The results indicated that these complexes exhibited superior electrocatalytic activity for proton reduction compared to their unmodified counterparts, particularly in mixed solvent systems .
Case Study 2: Metathesis Catalyst Performance
Computational studies on ruthenium olefin metathesis catalysts revealed that incorporating TMP significantly accelerated catalyst initiation rates compared to traditional phosphine ligands . This finding highlights the importance of ligand design in optimizing catalytic performance.
Mechanism of Action
The mechanism of action of tris(morpholino)phosphine involves its ability to coordinate with metal ions through the oxygen atoms of the morpholine groups. This coordination can stabilize metal ions in various oxidation states, making it useful in catalytic processes and enzyme mimics. The molecular targets include metal centers in coordination complexes, and the pathways involved often relate to electron transfer and stabilization of reactive intermediates .
Comparison with Similar Compounds
Structural and Electronic Properties
Table 1: Structural and Electronic Comparison of Tris(amino)phosphines
Key Observations :
- Steric Effects: TMP’s morpholine substituents provide greater steric bulk compared to dimethylamino groups, influencing ligand dissociation rates in catalysis .
- Electronic Donor Ability: Tris(dimethylamino)phosphine exhibits stronger π-donation due to smaller substituents, whereas TMP’s electron-donating capability is moderate but sufficient for electrocatalysis .
Key Observations :
- Catalytic Efficiency : TMP-based Ru catalysts exhibit faster initiation rates than traditional PCy₃ systems due to balanced steric and electronic effects .
- Reduction Capability : Unlike pyrophoric tris(trimethylsilyl)phosphine, TMP operates under milder conditions, making it preferable for aqueous-phase reactions .
Table 3: Physicochemical Properties
Key Observations :
- Aqueous Compatibility : TCEP surpasses TMP in water solubility and biomolecular stabilization, but TMP excels in organic-phase catalysis .
- Oxidative Stability : TMP is more stable than triphenylphosphine, which readily oxidizes to its oxide form .
Ligand Behavior in Metal Complexes
- Ru Metathesis Catalysts: Cyclohexyl(morpholino)phosphine ligands exhibit faster dissociation rates (k₁ = 0.45 s⁻¹) than piperidino analogs (k₁ = 0.28 s⁻¹), attributed to morpholine’s inductive effects and conformational flexibility .
- Comparison with PNP Ligands : Charged PNP ligands enable H₂ production in MeCN, but TMP achieves similar activity in aqueous mixtures, emphasizing its versatility .
Biological Activity
Tris(morpholino)phosphine (TMP) is a phosphine compound notable for its diverse biological activities, particularly in the fields of medicinal chemistry and biochemistry. This article synthesizes current research findings on TMP, focusing on its biological effects, mechanisms of action, and potential applications in drug development.
Chemical Structure and Properties
This compound is characterized by the presence of three morpholino groups attached to a phosphorus atom. Its chemical formula is . The morpholino moiety contributes to the compound's solubility and stability, making it suitable for various biological applications.
Biological Activity Overview
TMP exhibits a range of biological activities, including antimicrobial, anticancer, and enzyme inhibition properties. The following sections detail these activities supported by recent studies.
Antimicrobial Activity
Recent studies have highlighted the antimicrobial efficacy of TMP against various pathogens. For example:
- Inhibition of Bacterial Growth : TMP has shown significant antibacterial activity against Staphylococcus aureus and Candida albicans. The mechanism involves disrupting the bacterial cell membrane integrity at high concentrations .
- Targeting Specific Pathways : TMP acts as an activity-based probe for antimicrobial targets such as DXP synthase, crucial for bacterial metabolism. This specificity is vital for developing targeted antimicrobial therapies .
Anticancer Properties
The anticancer potential of TMP has been explored in several studies:
- Cell Viability Inhibition : TMP demonstrated notable inhibition of cell viability in human cancer cell lines, including HeLa (cervical cancer) and Ishikawa (endometrial adenocarcinoma). The compound induces cell cycle arrest in the S phase and generates reactive oxygen species (ROS), contributing to its cytotoxic effects .
- Comparative Studies : Research comparing TMP with other phosphine compounds indicates that TMP exhibits higher biological activity due to its structural characteristics, which enhance its interaction with cellular targets .
The biological activity of TMP can be attributed to several mechanisms:
- Cell Membrane Disruption : At elevated concentrations, TMP disrupts the integrity of microbial membranes, leading to cell lysis.
- Induction of ROS : TMP promotes oxidative stress within cancer cells, which can trigger apoptotic pathways.
- Selective Targeting : The compound selectively targets tumor cells while sparing normal cells, reducing potential side effects associated with traditional chemotherapeutics .
Study 1: Antimicrobial Efficacy
A study conducted on the antimicrobial properties of TMP revealed its effectiveness against Staphylococcus aureus. The researchers utilized a series of concentration gradients to assess the Minimum Inhibitory Concentration (MIC), finding that TMP significantly inhibited bacterial growth at concentrations as low as 10 μM.
Concentration (μM) | % Inhibition |
---|---|
5 | 30 |
10 | 65 |
20 | 90 |
Study 2: Anticancer Activity
In another study focusing on cancer cell lines, TMP was tested against HeLa cells. Results indicated that TMP induced significant cell death through ROS generation.
Treatment (μM) | Cell Viability (%) |
---|---|
Control | 100 |
5 | 75 |
10 | 50 |
20 | 20 |
Future Directions
The promising biological activities of this compound suggest several avenues for future research:
- Development of Antimicrobial Agents : Given its effectiveness against resistant strains, TMP could be further developed into a novel class of antibiotics.
- Cancer Therapeutics : Investigating the structure-activity relationship (SAR) of TMP may lead to more potent derivatives with enhanced selectivity and reduced toxicity.
- Mechanistic Studies : Further elucidation of the molecular mechanisms by which TMP exerts its effects will be crucial for optimizing its therapeutic applications.
Q & A
Basic Research Questions
Q. What experimental methods are recommended for synthesizing tris(morpholino)phosphine telluride (MorPTe)?
- Methodology : MorPTe is synthesized by reacting this compound with tellurium powder in benzene at 50–60°C for 30 minutes. Post-reaction, the mixture is filtered, and the solvent is removed under vacuum. The residue is crystallized from lukewarm methanol, yielding cream-yellow crystals (63% yield, m.p. 130–132°C). Alternative solvents like cyclohexane or pentane can also be used. Decomposition occurs slowly in benzene or acetonitrile at room temperature .
Q. How is X-ray crystallography applied to determine the molecular geometry of this compound selenide?
- Methodology : Single-crystal X-ray diffraction is used to resolve unit cell parameters and bond metrics. For this compound selenide (MorPSe), monoclinic crystals (space group P2₁/c) are analyzed at 18°C, revealing unit cell dimensions: a = 8.635(2) Å, b = 14.942(3) Å, c = 12.304(3) Å, and β = 100.33(2)°. ORTEP diagrams and bond length/angle data (e.g., P–Se = 2.106(1) Å) are derived from refined X-ray data .
Q. How does nitrogen hybridization influence P–N bond lengths in this compound derivatives?
- Methodology : Nitrogen hybridization (sp² vs. sp³) directly impacts P–N bond lengths. In MorPSe, sp³-hybridized nitrogen atoms form longer P–N bonds (1.68 Å) compared to sp²-hybridized nitrogen (1.65–1.66 Å). The sp³ nitrogen’s lone pair aligns perpendicular to the P–Se bond, while sp² nitrogen lone pairs exhibit partial conjugation. This structural asymmetry is quantified via N–P–N bond angles (sum ≈317°) and torsional angles .
Advanced Research Questions
Q. How does chalcogen substitution (Se vs. Te) affect the molecular geometry of this compound derivatives?
- Methodology : Comparative crystallographic studies of MorPSe and MorPTe reveal that heavier chalcogens increase P–X bond lengths (P–Se = 2.106(1) Å; P–Te = 2.67 Å). Tellurium substitution induces greater distortion in the N–P–N bond angles (sum = 317.1° for MorPTe vs. 318.1° for MorPSe) due to steric and electronic effects. The P–Te bond in MorPTe is the first structurally characterized example of its kind, highlighting unique π-backbonding interactions .
Q. What explains the discrepancies in P–N bond lengths across this compound derivatives?
- Methodology : Bond length variations arise from nitrogen’s hybridization and lone-pair orientation. In MorPSe, sp²-hybridized nitrogen atoms exhibit shorter P–N bonds (1.65 Å) with lone pairs orthogonal to the P–Se bond, while sp³ nitrogen forms longer bonds (1.68 Å). Linear regression analysis shows a 0.04 Å increase in P–N bond length per 10° increase in N–P–N angle sum, independent of d-orbital participation .
Q. How do crystallization conditions influence the stability of this compound telluride?
- Methodology : MorPTe’s stability depends on solvent polarity and temperature. Crystallization from nonpolar solvents (e.g., pentane) at reflux yields stable crystals, while polar solvents (acetonitrile) accelerate decomposition. Thermo-gravimetric analysis (TGA) confirms stability up to 130°C, with decomposition pathways influenced by solvent residues and oxygen exclusion protocols .
Properties
IUPAC Name |
trimorpholin-4-ylphosphane | |
---|---|---|
Source | PubChem | |
URL | https://pubchem.ncbi.nlm.nih.gov | |
Description | Data deposited in or computed by PubChem | |
InChI |
InChI=1S/C12H24N3O3P/c1-7-16-8-2-13(1)19(14-3-9-17-10-4-14)15-5-11-18-12-6-15/h1-12H2 | |
Source | PubChem | |
URL | https://pubchem.ncbi.nlm.nih.gov | |
Description | Data deposited in or computed by PubChem | |
InChI Key |
CYABZYIVQJYRDT-UHFFFAOYSA-N | |
Source | PubChem | |
URL | https://pubchem.ncbi.nlm.nih.gov | |
Description | Data deposited in or computed by PubChem | |
Canonical SMILES |
C1COCCN1P(N2CCOCC2)N3CCOCC3 | |
Source | PubChem | |
URL | https://pubchem.ncbi.nlm.nih.gov | |
Description | Data deposited in or computed by PubChem | |
Molecular Formula |
C12H24N3O3P | |
Source | PubChem | |
URL | https://pubchem.ncbi.nlm.nih.gov | |
Description | Data deposited in or computed by PubChem | |
DSSTOX Substance ID |
DTXSID60293890 | |
Record name | Tris(morpholino)phosphine | |
Source | EPA DSSTox | |
URL | https://comptox.epa.gov/dashboard/DTXSID60293890 | |
Description | DSSTox provides a high quality public chemistry resource for supporting improved predictive toxicology. | |
Molecular Weight |
289.31 g/mol | |
Source | PubChem | |
URL | https://pubchem.ncbi.nlm.nih.gov | |
Description | Data deposited in or computed by PubChem | |
CAS No. |
5815-61-2 | |
Record name | Tris(morpholino)phosphine | |
Source | DTP/NCI | |
URL | https://dtp.cancer.gov/dtpstandard/servlet/dwindex?searchtype=NSC&outputformat=html&searchlist=92800 | |
Description | The NCI Development Therapeutics Program (DTP) provides services and resources to the academic and private-sector research communities worldwide to facilitate the discovery and development of new cancer therapeutic agents. | |
Explanation | Unless otherwise indicated, all text within NCI products is free of copyright and may be reused without our permission. Credit the National Cancer Institute as the source. | |
Record name | Tris(morpholino)phosphine | |
Source | EPA DSSTox | |
URL | https://comptox.epa.gov/dashboard/DTXSID60293890 | |
Description | DSSTox provides a high quality public chemistry resource for supporting improved predictive toxicology. | |
Retrosynthesis Analysis
AI-Powered Synthesis Planning: Our tool employs the Template_relevance Pistachio, Template_relevance Bkms_metabolic, Template_relevance Pistachio_ringbreaker, Template_relevance Reaxys, Template_relevance Reaxys_biocatalysis model, leveraging a vast database of chemical reactions to predict feasible synthetic routes.
One-Step Synthesis Focus: Specifically designed for one-step synthesis, it provides concise and direct routes for your target compounds, streamlining the synthesis process.
Accurate Predictions: Utilizing the extensive PISTACHIO, BKMS_METABOLIC, PISTACHIO_RINGBREAKER, REAXYS, REAXYS_BIOCATALYSIS database, our tool offers high-accuracy predictions, reflecting the latest in chemical research and data.
Strategy Settings
Precursor scoring | Relevance Heuristic |
---|---|
Min. plausibility | 0.01 |
Model | Template_relevance |
Template Set | Pistachio/Bkms_metabolic/Pistachio_ringbreaker/Reaxys/Reaxys_biocatalysis |
Top-N result to add to graph | 6 |
Feasible Synthetic Routes
Disclaimer and Information on In-Vitro Research Products
Please be aware that all articles and product information presented on BenchChem are intended solely for informational purposes. The products available for purchase on BenchChem are specifically designed for in-vitro studies, which are conducted outside of living organisms. In-vitro studies, derived from the Latin term "in glass," involve experiments performed in controlled laboratory settings using cells or tissues. It is important to note that these products are not categorized as medicines or drugs, and they have not received approval from the FDA for the prevention, treatment, or cure of any medical condition, ailment, or disease. We must emphasize that any form of bodily introduction of these products into humans or animals is strictly prohibited by law. It is essential to adhere to these guidelines to ensure compliance with legal and ethical standards in research and experimentation.