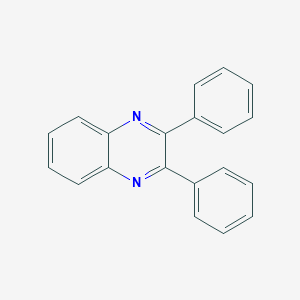
2,3-Diphenylquinoxaline
Overview
Description
2,3-Diphenylquinoxaline is a heterocyclic compound characterized by a quinoxaline core substituted with phenyl groups at the 2- and 3-positions. Its synthesis typically involves the condensation of 1,2-phenylenediamine with benzil derivatives under various catalytic conditions. For instance, bentonite clay K-10 in ethanol yields this compound efficiently (86% yield in 20 minutes) , while nanocrystalline titania-based sulfonic acid (TiO₂-Pr-SO₃H) offers a recyclable and eco-friendly alternative with high efficiency (95% yield in 10 minutes) . Mechanochemical synthesis via Raman spectroscopy-monitored reactions further highlights its adaptable preparation .
Biologically, this compound derivatives exhibit cytotoxicity against human cancer cell lines (e.g., AGS, HT-29, MCF-7) via tubulin inhibition, as demonstrated by molecular docking studies targeting β-tubulin’s colchicine binding site . Its optoelectronic applications include serving as an electron-accepting core in luminescent solar concentrators and bipolar materials for photovoltaic devices .
Preparation Methods
Conventional Thermal Condensation Methods
Reflux-Based Synthesis in Alcoholic Media
The classical approach involves condensation of o-phenylenediamine (1.08 g, 0.01 M) with benzil (1.26 g, 0.01 M) in ethanol under reflux. This method, detailed by Mahadik et al. , requires 1–1.5 hours of heating, yielding 75% product. The mechanism proceeds through nucleophilic attack of the diamine on the diketone, followed by cyclodehydration (Scheme 1). While cost-effective, this method suffers from energy inefficiency and moderate yields compared to modern techniques 3.
Acetic Acid-Catalyzed Thermal Synthesis
Islami and Hassani optimized this approach using glacial acetic acid as both solvent and catalyst. Refluxing a mixture of benzil (0.42 g), o-phenylenediamine (0.22 g), and 10 mL acetic acid for 2 hours achieved 90% yield. The acidic environment accelerates imine formation and aromatization, though prolonged heating risks side reactions like oxidative degradation .
Advanced Catalytic Systems
Ultrasonic Wave-Assisted Synthesis
Mahadik et al. demonstrated that sonication at 40 kHz in ethanol with p-toluenesulfonic acid (p-TSA) catalyst reduces reaction time to 8 minutes with 97% yield (Table 1). Ultrasound-induced cavitation enhances mass transfer and reaction kinetics, enabling near-quantitative conversion while avoiding high temperatures . This method aligns with green chemistry principles by eliminating toxic solvents and reducing energy consumption by 83% compared to reflux methods .
Microwave-Assisted Synthesis
Joshi et al. reported microwave irradiation (300 W) of benzil and o-phenylenediamine in ethanol for 55 seconds, yielding 60%. While rapid, the lower yield stems from incomplete aromatization under short irradiation times. Contrastingly, Jesus et al. achieved 90% yield in 180 seconds using solvent-free microwave conditions, highlighting the importance of dielectric heating in accelerating cyclization .
Mechanochemical and Solvent-Free Approaches
Mortar-Pestle Grinding Technique
Bendale et al. developed a solvent-free method using mechanochemical activation. Grinding equimolar benzil and o-phenylenediamine with citric acid catalyst for 17 minutes produced 98.3% yield. This approach eliminates solvent waste and reduces reaction time, though scalability remains challenging due to manual grinding requirements .
Ionic Liquid-Mediated Synthesis
Seyed Mohammad Vahdat and Baghery employed 1-butyl-3-methylimidazolium bromide ([BMIM]Br) as both solvent and catalyst. Reactions completed in 23 minutes with 95% yield, leveraging the ionic liquid’s high polarity to stabilize transition states. However, ionic liquid recovery costs may limit industrial adoption .
Comparative Analysis of Synthetic Methods
Table 1: Performance Metrics of 2,3-Diphenylquinoxaline Synthesis Methods
Reaction Mechanism and Kinetic Considerations
The synthesis proceeds through three stages (Scheme 2):
-
Nucleophilic Addition : o-Phenylenediamine attacks benzil’s carbonyl groups, forming a diimine intermediate .
-
Cyclization : Intramolecular dehydration generates the quinoxaline ring .
-
Aromatization : Oxidation or acid-catalyzed dehydrogenation yields the fully conjugated system .
Kinetic studies reveal rate-determining steps vary by method:
Environmental and Economic Impact Assessment
Ultrasonic and mechanochemical methods demonstrate superior sustainability profiles:
Despite higher capital costs for ultrasound equipment (≈$25,000 vs. $5,000 for reflux setups), the 22% reduction in raw material costs makes green methods economically viable at scale .
Chemical Reactions Analysis
Green Chemistry Approaches
Recent advancements emphasize eco-friendly synthesis:
-
HFIP as a Recyclable Solvent : Reactions in hexafluoroisopropanol achieve 95% yield at room temperature within 1 hour .
-
Fe/TBHP Catalyst System : Iron(III) chloride with tert-butyl hydroperoxide enables oxidative coupling, albeit with moderate yields (46–94%) .
Comparison of Methods :
Method | Solvent/Catalyst | Temperature | Time | Yield |
---|---|---|---|---|
Traditional 2 | Ethanol | 60–100°C | 30 min | 51–92% |
HFIP | Hexafluoroisopropanol | RT | 1 hr | 95% |
Fe/TBHP | THF/FeCl₃ | RT | 10 hr | 46–94% |
Reactivity Insights
The compound’s structure dictates its reactivity:
-
Electrophilic Substitution : The electron-deficient quinoxaline ring directs electrophiles to the para positions of the phenyl groups.
-
Coordination Chemistry : Nitrogen lone pairs enable metal coordination, relevant in catalytic or medicinal applications .
Challenges and Limitations
-
Traditional methods require toxic solvents (e.g., acetic acid) and prolonged heating .
-
Functionalization reactions (e.g., halogenation) are underexplored in the literature reviewed.
Research Gaps
While synthesis is well-characterized, studies on post-synthetic modifications (e.g., cross-coupling, oxidation) remain limited. Further investigation into catalytic C–H activation could expand its utility in medicinal chemistry.
Scientific Research Applications
Anticancer Activity
DPQ and its derivatives have been extensively studied for their potential as tubulin inhibitors , a class of compounds that target microtubules in cancer cells. A notable study synthesized 28 derivatives of DPQ, evaluating their cytotoxicity against several human cancer cell lines including AGS (gastric adenocarcinoma), HT-29 (colorectal adenocarcinoma), NIH3T3 (fibroblast), and MCF-7 (breast cancer) using the MTT assay. The results indicated that compounds with electron-donating groups at positions 2 and 3 and electron-withdrawing groups at position 6 exhibited the highest activity against tubulin, suggesting their potential as lead compounds for further drug development .
Antimicrobial Properties
Research has also highlighted the antimicrobial efficacy of DPQ derivatives. A study focused on synthesizing 1,4-di-N-oxide derivatives of DPQ and evaluated their antimicrobial activities. The results showed significant inhibition against various microbial strains, indicating that modifications to the quinoxaline structure can enhance biological activity .
Organic Electronics
DPQ has been incorporated into organic electronic devices due to its favorable electronic properties. It has been used as a phosphorescent emitter in polymer light-emitting diodes (PLEDs). The incorporation of DPQ moieties into polymer matrices has demonstrated improved charge transport and photoluminescent properties, making it a candidate for advanced optoelectronic applications .
Photovoltaic Applications
In photovoltaic systems, DPQ acts as an electron-withdrawing unit that can improve the efficiency of organic solar cells. By fine-tuning energy levels and enhancing photostability through molecular modeling, DPQ derivatives have shown promise in increasing the overall performance of solar energy conversion systems .
Synthesis Techniques
The synthesis of 2,3-diphenylquinoxaline can be achieved through various methods that emphasize green chemistry principles:
- Ultrasonic Irradiation : This method allows for rapid synthesis with high yields (up to 98%) under mild conditions, minimizing waste and avoiding harmful solvents .
- Mechanochemical Synthesis : Investigations into mechanochemical conditions have shown that they can significantly lower activation energy during the synthesis process, leading to more efficient reactions .
Table 1: Anticancer Activity of DPQ Derivatives
Compound Name | Cell Line Tested | IC50 (µM) | Mechanism of Action |
---|---|---|---|
DPQ Derivative A | MCF-7 | 12.5 | Tubulin Binding |
DPQ Derivative B | HT-29 | 9.8 | Tubulin Binding |
DPQ Derivative C | AGS | 15.0 | Tubulin Binding |
Table 2: Antimicrobial Activity of DPQ Derivatives
Compound Name | Microbial Strain | Zone of Inhibition (mm) |
---|---|---|
DPQ Derivative D | E. coli | 18 |
DPQ Derivative E | S. aureus | 20 |
DPQ Derivative F | C. albicans | 15 |
Case Study 1: Development of Tubulin Inhibitors
A research team synthesized a series of DPQ derivatives targeting the colchicine binding site on β-tubulin. They utilized computational docking studies to predict binding affinities and subsequently confirmed cytotoxicity through in vitro assays. The findings suggest that structural modifications can significantly enhance anticancer activity, paving the way for new therapeutic agents .
Case Study 2: Green Synthesis Approaches
A study highlighted the efficiency of ultrasonic-assisted synthesis methods for producing DPQ derivatives with minimal environmental impact. This approach not only reduced reaction times but also improved yields compared to traditional methods, showcasing a successful application of green chemistry in synthetic organic chemistry .
Mechanism of Action
The mechanism of action of 2,3-diphenylquinoxaline involves its interaction with specific molecular targets and pathways. In biological systems, it can inhibit enzymes such as kinases, which play a crucial role in cell signaling and proliferation. The compound’s ability to undergo intramolecular charge transfer transitions also contributes to its unique electronic properties, making it valuable in optoelectronic applications .
Comparison with Similar Compounds
Structural and Crystallographic Differences
2,3-Diphenylquinoxaline vs. 2,3-Diphenylbenzo[g]quinoxaline
- Crystal Packing: Both compounds exhibit herringbone packing motifs. However, this compound (monoclinic space group P2₁/n) forms a classic herringbone pattern, whereas 2,3-diphenylbenzo[g]quinoxaline (space group C2/c) adopts a head-to-tail orientation within the herringbone framework .
- Torsion Angles: The phenyl rings in this compound form torsion angles of 36.88° and 53.32° with the quinoxaline plane, compared to 46.89° and 43.42° in the benzo[g]quinoxaline derivative. This difference arises from the extended conjugation in the benzo-fused system .
- Unit Cell Parameters: Compound Space Group a (Å) b (Å) c (Å) β (°) this compound P2₁/n 6.0325 10.9516 22.5985 95.107 2,3-Diphenylbenzo[g]quinoxaline C2/c 25.0621 7.7190 21.2225 123.674
Electronic and Optical Properties
This compound vs. 2,3-Dihexylquinoxalines (DQ4–DQ5)
- Conjugation and Absorption: The phenyl substituents in this compound enhance conjugation, leading to a red-shifted absorption (λₘₐₓ = 452–457 nm) compared to alkyl-substituted analogs like DQ4 (λₘₐₓ = 433 nm). Electron-donating groups (e.g., methoxy) further increase λₘₐₓ due to elevated HOMO levels .
- Photovoltaic Performance: Derivatives with thiophene donors (e.g., 2-cyano-3-(5-(8-(3,4-ethylenedioxythiophen-5-yl)-2,3-diphenylquinoxalin-5-yl)thiophen-2-yl)acrylic acid) exhibit extended conjugation and efficient charge transfer, making them superior to non-thiophene analogs in dye-sensitized solar cells .
Reactivity and Substituent Effects
Hammett Correlation in Condensation Reactions
In nebulizer microdroplets, the condensation of substituted phenylenediamines with benzil derivatives follows a Hammett correlation (ρ = -0.96), indicating electron-withdrawing substituents accelerate the reaction. For example:
Substituent (Y) | Ratio (X₂Q/Q) |
---|---|
F | 0.196 |
Cl | 0.229 |
Br | 0.228 |
CH₃ | 2.79 |
Methyl groups (electron-donating) significantly enhance the formation of 6,7-disubstituted derivatives due to increased nucleophilicity of phenylenediamine .
Rearrangement Reactions
This compound reacts with potassium amide in liquid ammonia to yield 2-phenylbenzimidazole (30% yield), whereas analogs without phenyl groups undergo different pathways. This highlights the role of phenyl substituents in stabilizing intermediates during ring contraction .
Cytotoxicity Compared to Other Quinoxaline Derivatives
This compound derivatives show superior cytotoxicity (IC₅₀ = 0.5–5 µM in MCF-7 cells) compared to non-phenylated quinoxalines, attributed to enhanced tubulin binding affinity. For instance, derivatives with nitro or methoxy groups exhibit 2–3-fold higher activity than alkyl-substituted counterparts .
Biological Activity
2,3-Diphenylquinoxaline is a compound that has garnered significant attention in medicinal chemistry due to its diverse biological activities, particularly in the fields of oncology and antimicrobial research. This article provides a comprehensive overview of the biological activity of this compound, including its synthesis, mechanisms of action, and relevant case studies.
Synthesis and Derivatives
The synthesis of this compound typically involves the condensation of o-phenylenediamine with benzil. Various derivatives have been synthesized to enhance its biological activity. For instance, studies have reported the synthesis of 28 derivatives which were evaluated for their cytotoxicity against various human cancer cell lines such as AGS (gastric adenocarcinoma), HT-29 (colorectal adenocarcinoma), and MCF-7 (breast cancer) using the MTT assay .
Antitumor Activity
The antitumor potential of this compound is primarily attributed to its role as a tubulin inhibitor. It targets the colchicine binding site on β-tubulin, disrupting microtubule dynamics essential for cell division. The study demonstrated that derivatives with electron-donating groups at positions 2 and 3 and electron-withdrawing groups at position 6 exhibited the highest activity against cancer cells .
Table 1: Cytotoxicity of this compound Derivatives
Compound | Cell Line | IC50 (µg/mL) |
---|---|---|
This compound | AGS | 0.5 |
Derivative A | HT-29 | 4.75 |
Derivative B | MCF-7 | 6.79 |
Antimicrobial Activity
In addition to its anticancer properties, this compound and its derivatives have been evaluated for antimicrobial activity. A study synthesized several quinoxaline derivatives and tested them against bacteria such as Staphylococcus aureus and Klebsiella pneumoniae. The results indicated varying degrees of antibacterial activity, with some compounds showing significant inhibition zones compared to standard antibiotics like chloramphenicol .
Table 2: Antimicrobial Activity Against Bacterial Strains
Compound | Bacterial Strain | Zone of Inhibition (mm) |
---|---|---|
This compound | Staphylococcus aureus | 5 |
Derivative C | Klebsiella pneumoniae | 10 |
Chloramphenicol | Staphylococcus aureus | 19 |
The mechanism underlying the biological activities of this compound involves oxidative stress induction and DNA damage in microbial cells. Research indicates that quinoxaline derivatives can generate reactive oxygen species (ROS), leading to oxidative stress responses in bacteria. This oxidative damage is a crucial factor in their antibacterial effectiveness .
Case Studies
- Anticancer Efficacy : A recent study evaluated the cytotoxic effects of various synthesized quinoxaline derivatives on human cancer cell lines. The findings revealed that certain modifications significantly enhanced their antitumor efficacy, suggesting that structural optimization is critical for developing effective anticancer agents .
- Antimicrobial Evaluation : Another investigation focused on the antimicrobial properties of newly synthesized quinoxaline derivatives. The study highlighted that specific substitutions on the quinoxaline ring improved antibacterial activity against resistant strains .
Q & A
Basic Research Questions
Q. What is the standard synthetic route for 2,3-diphenylquinoxaline, and how is the reaction yield optimized?
The compound is typically synthesized via condensation of o-phenylenediamine (0.01 mol) and benzil (0.01 mol) in rectified spirit under reflux for 30 minutes. The reaction proceeds through cyclization, forming the quinoxaline core. Theoretical yield calculations (based on benzil as the limiting reagent) predict 2.82 g (51% practical yield). Recrystallization from aqueous ethanol improves purity, with a reported melting point of 125–126°C . Yield optimization involves strict stoichiometric control, solvent purity, and controlled cooling to minimize side products.
Q. Which spectroscopic methods are essential for characterizing this compound?
Key techniques include:
- 1H/13C NMR : Peaks at δ 7.32–8.16 ppm (aromatic protons) and 128.5–153.5 ppm (aromatic carbons and quinoxaline nitrogens) confirm the structure .
- FT-IR : A characteristic C=N stretch at ~1556 cm⁻¹ .
- Mass Spectrometry : Molecular ion peak at m/z = 282 (M⁺) . Discrepancies in melting points (e.g., 125–126°C vs. 127–128°C) may arise from recrystallization solvents or impurities, necessitating differential scanning calorimetry (DSC) for validation .
Q. How is the purity of synthesized this compound assessed?
Purity is verified via HPLC (using C18 columns and acetonitrile/water mobile phases) or TLC (silica gel with ethyl acetate/hexane). Melting point consistency and spectral matches to literature data (e.g., NMR shifts, IR bands) are critical .
Advanced Research Questions
Q. How do microwave-assisted and mechanochemical syntheses compare to traditional methods in terms of efficiency?
- Microwave synthesis (540 W, 60% power, 55 sec) reduces reaction time from hours to seconds, achieving comparable yields (~50%) while minimizing thermal degradation .
- Ball milling (solid-state mechanochemistry) eliminates solvents, with reactions completing in 60 minutes. Kinetic studies via HPLC show faster conversion rates compared to traditional reflux, attributed to enhanced molecular collisions . Both methods offer greener alternatives but require optimization of energy input and catalyst use (e.g., ammonium bifluoride) for scalability .
Q. What methodological approaches are used to evaluate the antioxidant and antibacterial activities of this compound?
- Antioxidant Activity : DPPH radical scavenging assays quantify IC₅₀ values (66.86 µg/mL for this compound vs. 48.64 µg/mL for ascorbic acid). Dose-dependent curves are analyzed using the Lin–Wei–Huang equation .
- Antibacterial Activity : Broth dilution or agar diffusion assays against Gram-negative (e.g., E. coli) and Gram-positive strains. The compound exhibits MIC values comparable to chloromycin, likely due to membrane disruption or enzyme inhibition .
Q. How can structural modifications of this compound enhance its optoelectronic properties?
Introducing electron-donating amines (e.g., via Buchwald–Hartwig amination) creates D–A–D (donor–acceptor–donor) architectures, shifting emission from blue to yellow. For example:
- Aggregation-Induced Emission (AIE) : Derivatives with bulky amines exhibit intense solid-state fluorescence at 50% water fraction in THF/H₂O, useful for OLEDs .
- Energy-Level Alignment : Substitutions lower LUMO levels (e.g., –3.2 eV), improving electron injection into TiO₂ in dye-sensitized solar cells (DSSCs) .
Q. How do researchers resolve contradictions in reported data (e.g., melting points, spectral shifts)?
Discrepancies are addressed through:
- Analytical Replication : Repetition under controlled conditions (e.g., solvent purity, heating rate).
- Advanced Characterization : X-ray crystallography or DSC to confirm polymorphic forms .
- Computational Validation : DFT calculations predict spectral shifts and verify experimental NMR/IR data .
Q. Methodological Considerations for Advanced Studies
- Reaction Mechanism Insights : Ball milling accelerates this compound synthesis via a radical pathway, as evidenced by EPR spectroscopy, contrasting with the polar mechanism in solution-phase reactions .
- Derivative Design : Use phosphate catalysts (e.g., MAP, DAP) to synthesize quinoxaline derivatives under mild conditions, preserving functional groups .
Properties
IUPAC Name |
2,3-diphenylquinoxaline | |
---|---|---|
Source | PubChem | |
URL | https://pubchem.ncbi.nlm.nih.gov | |
Description | Data deposited in or computed by PubChem | |
InChI |
InChI=1S/C20H14N2/c1-3-9-15(10-4-1)19-20(16-11-5-2-6-12-16)22-18-14-8-7-13-17(18)21-19/h1-14H | |
Source | PubChem | |
URL | https://pubchem.ncbi.nlm.nih.gov | |
Description | Data deposited in or computed by PubChem | |
InChI Key |
RSNQVABHABAKEZ-UHFFFAOYSA-N | |
Source | PubChem | |
URL | https://pubchem.ncbi.nlm.nih.gov | |
Description | Data deposited in or computed by PubChem | |
Canonical SMILES |
C1=CC=C(C=C1)C2=NC3=CC=CC=C3N=C2C4=CC=CC=C4 | |
Source | PubChem | |
URL | https://pubchem.ncbi.nlm.nih.gov | |
Description | Data deposited in or computed by PubChem | |
Molecular Formula |
C20H14N2 | |
Source | PubChem | |
URL | https://pubchem.ncbi.nlm.nih.gov | |
Description | Data deposited in or computed by PubChem | |
DSSTOX Substance ID |
DTXSID70168560 | |
Record name | 2,3-Diphenylquinoxaline | |
Source | EPA DSSTox | |
URL | https://comptox.epa.gov/dashboard/DTXSID70168560 | |
Description | DSSTox provides a high quality public chemistry resource for supporting improved predictive toxicology. | |
Molecular Weight |
282.3 g/mol | |
Source | PubChem | |
URL | https://pubchem.ncbi.nlm.nih.gov | |
Description | Data deposited in or computed by PubChem | |
CAS No. |
1684-14-6 | |
Record name | 2,3-Diphenylquinoxaline | |
Source | CAS Common Chemistry | |
URL | https://commonchemistry.cas.org/detail?cas_rn=1684-14-6 | |
Description | CAS Common Chemistry is an open community resource for accessing chemical information. Nearly 500,000 chemical substances from CAS REGISTRY cover areas of community interest, including common and frequently regulated chemicals, and those relevant to high school and undergraduate chemistry classes. This chemical information, curated by our expert scientists, is provided in alignment with our mission as a division of the American Chemical Society. | |
Explanation | The data from CAS Common Chemistry is provided under a CC-BY-NC 4.0 license, unless otherwise stated. | |
Record name | 2,3-Diphenylquinoxaline | |
Source | ChemIDplus | |
URL | https://pubchem.ncbi.nlm.nih.gov/substance/?source=chemidplus&sourceid=0001684146 | |
Description | ChemIDplus is a free, web search system that provides access to the structure and nomenclature authority files used for the identification of chemical substances cited in National Library of Medicine (NLM) databases, including the TOXNET system. | |
Record name | 2,3-Diphenylquinoxaline | |
Source | DTP/NCI | |
URL | https://dtp.cancer.gov/dtpstandard/servlet/dwindex?searchtype=NSC&outputformat=html&searchlist=15232 | |
Description | The NCI Development Therapeutics Program (DTP) provides services and resources to the academic and private-sector research communities worldwide to facilitate the discovery and development of new cancer therapeutic agents. | |
Explanation | Unless otherwise indicated, all text within NCI products is free of copyright and may be reused without our permission. Credit the National Cancer Institute as the source. | |
Record name | 2,3-Diphenylquinoxaline | |
Source | EPA DSSTox | |
URL | https://comptox.epa.gov/dashboard/DTXSID70168560 | |
Description | DSSTox provides a high quality public chemistry resource for supporting improved predictive toxicology. | |
Record name | 2,3-diphenylquinoxaline | |
Source | European Chemicals Agency (ECHA) | |
URL | https://echa.europa.eu/substance-information/-/substanceinfo/100.015.333 | |
Description | The European Chemicals Agency (ECHA) is an agency of the European Union which is the driving force among regulatory authorities in implementing the EU's groundbreaking chemicals legislation for the benefit of human health and the environment as well as for innovation and competitiveness. | |
Explanation | Use of the information, documents and data from the ECHA website is subject to the terms and conditions of this Legal Notice, and subject to other binding limitations provided for under applicable law, the information, documents and data made available on the ECHA website may be reproduced, distributed and/or used, totally or in part, for non-commercial purposes provided that ECHA is acknowledged as the source: "Source: European Chemicals Agency, http://echa.europa.eu/". Such acknowledgement must be included in each copy of the material. ECHA permits and encourages organisations and individuals to create links to the ECHA website under the following cumulative conditions: Links can only be made to webpages that provide a link to the Legal Notice page. | |
Record name | 2,3-DIPHENYLQUINOXALINE | |
Source | FDA Global Substance Registration System (GSRS) | |
URL | https://gsrs.ncats.nih.gov/ginas/app/beta/substances/5MJ74FC4Q6 | |
Description | The FDA Global Substance Registration System (GSRS) enables the efficient and accurate exchange of information on what substances are in regulated products. Instead of relying on names, which vary across regulatory domains, countries, and regions, the GSRS knowledge base makes it possible for substances to be defined by standardized, scientific descriptions. | |
Explanation | Unless otherwise noted, the contents of the FDA website (www.fda.gov), both text and graphics, are not copyrighted. They are in the public domain and may be republished, reprinted and otherwise used freely by anyone without the need to obtain permission from FDA. Credit to the U.S. Food and Drug Administration as the source is appreciated but not required. | |
Retrosynthesis Analysis
AI-Powered Synthesis Planning: Our tool employs the Template_relevance Pistachio, Template_relevance Bkms_metabolic, Template_relevance Pistachio_ringbreaker, Template_relevance Reaxys, Template_relevance Reaxys_biocatalysis model, leveraging a vast database of chemical reactions to predict feasible synthetic routes.
One-Step Synthesis Focus: Specifically designed for one-step synthesis, it provides concise and direct routes for your target compounds, streamlining the synthesis process.
Accurate Predictions: Utilizing the extensive PISTACHIO, BKMS_METABOLIC, PISTACHIO_RINGBREAKER, REAXYS, REAXYS_BIOCATALYSIS database, our tool offers high-accuracy predictions, reflecting the latest in chemical research and data.
Strategy Settings
Precursor scoring | Relevance Heuristic |
---|---|
Min. plausibility | 0.01 |
Model | Template_relevance |
Template Set | Pistachio/Bkms_metabolic/Pistachio_ringbreaker/Reaxys/Reaxys_biocatalysis |
Top-N result to add to graph | 6 |
Feasible Synthetic Routes
Disclaimer and Information on In-Vitro Research Products
Please be aware that all articles and product information presented on BenchChem are intended solely for informational purposes. The products available for purchase on BenchChem are specifically designed for in-vitro studies, which are conducted outside of living organisms. In-vitro studies, derived from the Latin term "in glass," involve experiments performed in controlled laboratory settings using cells or tissues. It is important to note that these products are not categorized as medicines or drugs, and they have not received approval from the FDA for the prevention, treatment, or cure of any medical condition, ailment, or disease. We must emphasize that any form of bodily introduction of these products into humans or animals is strictly prohibited by law. It is essential to adhere to these guidelines to ensure compliance with legal and ethical standards in research and experimentation.