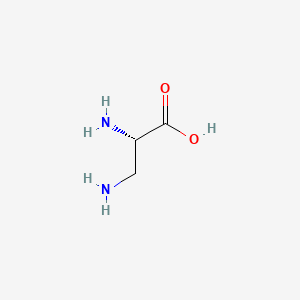
L-2,3-Diaminopropionic acid
Overview
Description
L-2,3-Diaminopropionic acid (L-Dap) is a nonproteinogenic diamino acid with the molecular formula C₃H₈N₂O₂ and a molecular weight of 104.11 g/mol . It features two amino groups on the second and third carbon atoms of a three-carbon backbone, distinguishing it from canonical amino acids. L-Dap is biosynthesized in Staphylococcus aureus via the enzymes SbnA (a cysteine synthase homolog) and SbnB (resembling amino acid dehydrogenases), which collectively act as an L-Dap synthase . Additionally, L-Dap serves as a precursor for antibiotics and is incorporated into synthetic peptides to modulate biological activity and stability .
Preparation Methods
Synthetic Chemical Preparation Methods
Reductive Amination of Protected d-Serine Derivatives
A prominent synthetic approach to L-Dap involves starting from optically pure protected d-serine derivatives. This method preserves stereochemistry and allows for orthogonal protection of amino groups, facilitating further chemical modifications.
-
- Starting material: Fmoc-O-tert-butyl-d-serine.
- Conversion to aldehyde derivative.
- Reductive amination with primary amines or sulfonamides, catalyzed by titanium isopropoxide (Ti(OiPr)4).
- Oxidation of the resulting 2,3-diaminopropanol intermediate to install the carboxyl group.
- Methylation of the carboxyl group to obtain methyl esters of L-Dap.
-
- Preservation of chirality from d-serine.
- Use of orthogonal protecting groups such as Fmoc, Boc, and tosyl (Ts) allows selective deprotection.
- Mild oxidation conditions (e.g., using trichloroisocyanuric acid (TCCA) with TEMPO catalyst) minimize side reactions.
- The process minimizes chromatographic purification steps, enhancing efficiency.
-
- Reductive amination yields range from 82% to 92% depending on the amine used (see Table 1 below).
- Overall yields for the methyl ester products are excellent, often obtained without chromatography.
Table 1: Yields of Reductive Amination Products in L-Dap Synthesis
Reagent (Amine) | Product Code | Yield (%) |
---|---|---|
Primary amine 4 | 4 | 92 |
Primary amine 5 | 5 | 90 |
Primary amine 6 | 6 | 90 |
Primary amine 7 | 7 | 91 |
n-Propylamine | 8 | 89 |
Primary amine 9 | 9 | 82 |
Primary amine 10 | 10 | 85 |
(Yields are isolated yields after chromatographic purification)
- Reference: Temperini et al. (2020) developed this method, emphasizing its applicability in peptide synthesis and alkylation chemistry.
Protection Strategies and Derivative Synthesis
- The use of α-Boc and β-Cbz protecting groups has been explored to improve yields and simplify purification.
- A synthetic route involving the formation of Boc-5-oxazolidone intermediates from N-Boc-L-aspartic acid and paraformaldehyde has been patented, offering a cost-effective and higher-yield alternative.
- This method involves catalytic tosic acid-mediated dehydration and subsequent vinyl chloroformate reaction steps to yield protected L-Dap derivatives.
- Advantages include fewer protection/deprotection steps and the use of less expensive reagents compared to other methods.
Biosynthetic Preparation of L-2,3-Diaminopropionic Acid
Enzymatic Biosynthesis via Microbial Pathways
- L-Dap is biosynthesized naturally in certain bacteria, including Staphylococcus aureus and Streptomyces species.
- The biosynthesis involves the conversion of L-serine to L-Dap through a pyridoxal phosphate-dependent β-replacement reaction.
- Mechanistically, the α-hydrogen of serine is eliminated, β-hydrogens are retained, and ammonia is added to the β-carbon with retention of stereochemistry.
- Enzymes SbnA and SbnB encoded in the staphyloferrin B biosynthetic gene cluster catalyze this process:
- SbnA uses O-phospho-L-serine and L-glutamate with PLP to form an intermediate metabolite.
- SbnB oxidatively hydrolyzes this intermediate to yield L-Dap and α-ketoglutarate.
- Crystal structures of these enzymes have elucidated substrate binding and catalytic residues, providing insight into enzymatic specificity and mechanism.
Novel Nonribosomal Peptide Synthetase (NRPS) Involvement
- In Streptomyces albulus, L-Dap is synthesized by two proteins homologous to cysteine synthetase and ornithine cyclodeaminase.
- L-Dap is then polymerized into poly(L-Dap) by a unique NRPS with adenylation, peptidyl carrier protein, and multiple transmembrane domains.
- This NRPS structure is unusual compared to classical NRPSs and resembles poly(ε-L-lysine) synthetase.
- Genetic and biochemical studies of this pathway offer potential for biotechnological production of L-Dap and its polymers.
Comparative Summary of Preparation Methods
Method Type | Starting Material | Key Features | Yield/Outcome | Applications |
---|---|---|---|---|
Synthetic Reductive Amination | Fmoc-O-tert-butyl-d-serine | Orthogonal protection, mild oxidation, high stereochemical control | 82-92% yields for intermediates; excellent overall | Peptide synthesis, alkylation, cyclization |
Protection Strategy via Boc-5-Oxazolidone | N-Boc-L-aspartic acid + paraformaldehyde | Cost-effective, fewer steps, catalytic dehydration | Higher yields, simpler purification | Protected L-Dap derivatives |
Enzymatic Biosynthesis | L-serine (microbial) | PLP-dependent β-replacement, enzyme catalysis | Natural biosynthesis, stereospecific | Antibiotic precursor, siderophore biosynthesis |
NRPS-mediated Biosynthesis | L-Dap monomers in Streptomyces | Novel NRPS with transmembrane domains | Polymerization into poly(L-Dap) | Biopolymer production |
Research Findings and Practical Considerations
- The synthetic methods offer scalable routes to L-Dap with high stereochemical fidelity, crucial for pharmaceutical and biochemical applications.
- Orthogonal protecting groups enable selective functionalization, facilitating the synthesis of derivatives and incorporation into peptides.
- Biosynthetic pathways provide insights for metabolic engineering and biotechnological production, potentially reducing costs and environmental impact.
- The enzymatic mechanisms elucidated by structural biology help in designing enzyme variants for tailored synthesis.
- Novel NRPS systems open avenues for producing homopolymers of L-Dap with unique properties.
Chemical Reactions Analysis
Types of Reactions: Diaminopropanoic acid undergoes various chemical reactions, including oxidation, reduction, and substitution. The compound’s amino groups make it reactive towards electrophiles, allowing for the formation of amides and other derivatives .
Common Reagents and Conditions: Common reagents used in reactions involving diaminopropanoic acid include pyridoxal phosphate for amination, titanium isopropoxide for reductive amination, and oxidizing agents like trichloroisocyanuric acid for carboxyl group installation .
Major Products: The major products formed from reactions involving diaminopropanoic acid include various protected derivatives, such as Fmoc-protected nucleoamino acids, which are used in the synthesis of nucleopeptides .
Scientific Research Applications
Biochemical Applications
1.1. Synthesis of Peptidomimetics
L-Dap is utilized in the synthesis of biotinylated peptidomimetics that enhance enzyme delivery into cells. These compounds facilitate the transport of active proteins, particularly enzymes, across cellular membranes. Studies have shown that L-Dap-based polymers can effectively deliver enzymes like β-galactosidase inside cells, demonstrating high compatibility and activity levels compared to control groups .
1.2. Gene Delivery Systems
Research has demonstrated that L-Dap can enhance nucleic acid delivery systems. By forming non-covalent complexes with nucleic acids, L-Dap facilitates their protection from degradation and promotes cellular uptake through endocytosis. The pH-responsive nature of L-Dap-rich peptides allows for effective gene silencing in various cell lines while maintaining low cytotoxicity .
Pharmacological Applications
2.1. Antibiotic Precursors
L-Dap serves as a precursor for biosynthesis of antibiotics and siderophores, such as staphyloferrin B produced by Staphylococcus aureus. The enzymatic pathways involving L-Dap are crucial for the survival and virulence of this pathogen, making it a target for antibiotic development .
2.2. Metabolic Stress Research
Studies have indicated that L-Dap accumulation can induce metabolic stress in bacteria like Salmonella enterica. The compound disrupts normal metabolic processes, leading to growth inhibition unless detoxified by specific enzymes such as diaminopropionate ammonia-lyase (DpaL). This insight is valuable for understanding bacterial metabolism and developing strategies to combat bacterial infections .
Environmental and Ecological Research
3.1. Plant Metabolites
L-Dap is also identified as a multifunctional metabolite in certain plants, notably in drought-tolerant species like the grass pea (Lathyrus sativus). While it contributes to plant resilience, excessive consumption can lead to neurolathyrism in humans, showcasing the dual nature of its effects .
Case Studies
Mechanism of Action
The mechanism by which diaminopropanoic acid exerts its effects involves its interaction with various enzymes and molecular targets. For example, in the biosynthesis of staphyloferrin B, diaminopropanoic acid is synthesized by enzymes SbnA and SbnB, which function together as an L-Dap synthase . This process is crucial for the iron-restricted growth of Staphylococcus aureus .
Comparison with Similar Compounds
L-Dap belongs to a family of diamino acids with varying side-chain lengths and structural configurations. Below is a systematic comparison with key analogs:
Structural and Chemical Properties
Key Observations :
- Side-Chain Length : L-Dap’s shorter side chain reduces steric bulk, enabling closer nucleobase positioning in nucleopeptides compared to L-Dab or L-Orn .
- Biosynthesis: Unlike L-Lys (synthesized via diaminopimelate pathway) or L-Orn (urea cycle), L-Dap is produced through a unique pathway involving oxidative hydrolysis of intermediates like 33N-(1-amino-1-carboxyl-2-ethyl)-glutamic acid .
Functional Contrasts :
- Siderophore Specificity : Staphyloferrin B uniquely requires L-Dap for iron binding, while other siderophores (e.g., aerobactin) use citrate or hydroxamates .
Research Findings and Challenges
- Biosynthetic Challenges: L-Dap’s nonproteinogenic nature complicates its large-scale production, requiring heterologous expression of sbnA and sbnB in engineered hosts .
- Structural Limitations : The short side chain of L-Dap restricts its utility in enzymes requiring longer substrates (e.g., PddB) .
- Therapeutic Potential: L-Dap-containing polymers (DAPEGs) show promise in gene delivery but require optimization for target specificity .
Biological Activity
L-2,3-Diaminopropionic acid (L-Dap) is a non-proteinogenic amino acid that plays significant roles in various biological processes, particularly in microbial metabolism and antibiotic biosynthesis. This article reviews the biological activity of L-Dap, with a focus on its metabolic functions, biosynthetic pathways, and implications in microbial physiology.
This compound is synthesized through the pyridoxal phosphate (PLP)-mediated amination of serine. It is recognized as a precursor for several biologically active compounds, including the siderophore staphyloferrin B produced by Staphylococcus aureus .
Chemical Structure:
- IUPAC Name: (2S)-2,3-diaminopropanoic acid
- Molecular Formula: C₃H₈N₂O₂
- Monoisotopic Molecular Weight: 104.05857751 g/mol
- CAS Registry Number: 4033-39-0
2.1 Role in Antibiotic Production
L-Dap serves as a critical building block in the synthesis of antibiotics and other secondary metabolites. For instance, it is involved in the biosynthesis of zwittermicin A, an antibiotic produced by Bacillus cereus .
2.2 Metabolic Stress and Growth Regulation
Research indicates that accumulation of L-Dap can induce metabolic stress in bacteria such as Salmonella enterica. Specifically, it has been shown to inhibit coenzyme A and isoleucine biosynthesis, leading to a requirement for proline for growth . The enzyme diaminopropionate ammonia-lyase (DpaL) facilitates the degradation of L-Dap into pyruvate and ammonia, alleviating this stress .
3.1 Staphyloferrin B Synthesis
A study demonstrated that mutations in genes encoding enzymes SbnA and SbnB disrupt the synthesis of staphyloferrin B. Supplementation with L-Dap restored growth in mutant strains, highlighting its essential role in iron acquisition under nutrient-limited conditions .
3.2 Metabolic Pathways Affected by L-Dap
The following table summarizes key metabolic pathways influenced by L-Dap:
Pathway | Effect of L-Dap | Reference |
---|---|---|
Coenzyme A Biosynthesis | Inhibition leading to growth requirement for proline | |
Isoleucine Biosynthesis | Direct inhibition | |
Staphyloferrin B Production | Essential for synthesis |
The mechanisms through which L-Dap exerts its biological effects involve:
- Siderophore Production: Facilitates iron uptake in bacteria.
- Metabolic Inhibition: Alters amino acid biosynthesis pathways leading to growth inhibition under certain conditions.
- Detoxification Pathways: Enzymatic degradation via DpaL helps mitigate toxic effects associated with high concentrations of L-Dap .
5.
This compound is a crucial amino acid with diverse biological activities ranging from antibiotic synthesis to regulation of metabolic pathways in bacteria. Its role in microbial physiology underscores its importance not only as a building block for secondary metabolites but also as a factor influencing bacterial growth and survival under stress conditions.
Future research should continue to explore the intricate biochemical pathways associated with L-Dap and its potential applications in biotechnology and medicine. Understanding these mechanisms could lead to novel strategies for combating bacterial infections or enhancing antibiotic production.
Q & A
Basic Research Questions
Q. What are the primary biosynthetic pathways for L-2,3-diaminopropionic acid (L-Dap) in microbial systems?
L-Dap is synthesized via enzyme-catalyzed reactions involving precursors like L-glutamate, L-threonine, or L-proline. In Staphylococcus aureus, the SbnA and SbnB enzymes are critical: SbnB acts as a dehydrogenase/cyclodeaminase, converting L-glutamate to L-Dap via α-ketoglutarate intermediates . Methodologically, gene knockout studies (e.g., sbnA/B mutants) combined with isotopic labeling (e.g., -glutamate) can track precursor incorporation and validate pathway steps .
Q. How can L-Dap be quantified in bacterial cultures or biological samples?
Reverse-phase HPLC coupled with UV/fluorescence detection is commonly used. Derivatization with ninhydrin or o-phthalaldehyde enhances sensitivity for amino acid quantification. For metabolic studies, stable isotope-labeled L-Dap (e.g., -labeled) can be traced via LC-MS .
Q. What role does L-Dap play in siderophore biosynthesis?
L-Dap is a key iron-chelating component in staphyloferrin B, a siderophore critical for iron acquisition in S. aureus. Its dual amine groups coordinate Fe(III), enabling microbial iron scavenging under nutrient-limited conditions. Disruption of sbnA/B genes abolishes siderophore production, which can be rescued by exogenous L-Dap supplementation .
Advanced Research Questions
Q. How do SbnA and SbnB enzymes mechanistically collaborate in L-Dap synthesis?
SbnA, a cysteine synthase homolog, generates a persulfide intermediate, while SbnB catalyzes dehydrogenation and cyclodeamination. Structural studies (e.g., X-ray crystallography at 2.11 Å resolution) reveal SbnB’s active site binds NAD and PLP, facilitating α-ketoglutarate release and L-Dap formation . Mutagenesis of conserved residues (e.g., His276 in SbnB) disrupts activity, validated via enzymatic assays with purified proteins .
Q. What strategies optimize L-Dap production in engineered microbial systems?
Heterologous expression of sbnA/B in E. coli enables L-Dap biosynthesis. Yield improvements involve:
- Overexpression of glutamate transporters (e.g., gltS) to enhance precursor supply.
- Knockout of competing pathways (e.g., thrB for threonine metabolism).
- Fed-batch fermentation with controlled nitrogen sources (e.g., ammonium sulfate) .
Q. How do L-Dap-containing peptides influence structural stability and bioactivity?
L-Dap’s dual amine groups enable unique hydrogen-bonding networks. In peptidomimetics, replacing lysine with L-Dap enhances proteolytic resistance and binding specificity. Circular dichroism (CD) and NMR studies show L-Dap incorporation alters peptide helicity, as seen in conotoxin analogs . For example, L-Dap substitution in tachykinin receptors improves fluorescence probe stability .
Q. What contradictions exist in L-Dap’s biosynthetic models, and how are they resolved?
Isotopic labeling in Streptomyces alanosinicus suggests L-Dap is loaded onto a peptidyl carrier protein (PCP) for stability during diazeniumdiolate biosynthesis . However, S. aureus studies propose direct incorporation into siderophores without PCP mediation . Comparative genomics and in vitro reconstitution assays (e.g., testing PCP-L-Dap interactions) are used to reconcile these models .
Q. Methodological Focus
Q. How are L-Dap derivatives synthesized for peptide chemistry applications?
Protected derivatives like N-Boc-N-allyloxycarbonyl-L-Dap are synthesized via stepwise protection:
- Boc-group (tert-butoxycarbonyl) for α-amine protection.
- Allyloxycarbonyl (Aloc) for β-amine protection.
- Deprotection via Pd-catalyzed allyl cleavage enables selective functionalization. Purity is confirmed by NMR and LC-MS .
Q. What analytical techniques characterize L-Dap-enzyme interactions?
- X-ray crystallography : Resolves SbnB-L-Dap binding modes (e.g., PDB: 4QYZ) .
- Isothermal titration calorimetry (ITC) : Measures binding affinity () between L-Dap and siderophore synthetases.
- Molecular dynamics simulations : Predict conformational changes during catalysis .
Q. How can L-Dap’s role in antibiotic resistance be investigated?
Iron-restricted growth assays with S. aureus mutants (ΔsbnA/B) quantify staphyloferrin B dependence. Co-culturing with competing microbes (e.g., Pseudomonas aeruginosa) tests L-Dap’s role in iron competition. Transcriptomics (RNA-seq) identifies L-Dap-regulated virulence genes .
Properties
IUPAC Name |
(2S)-2,3-diaminopropanoic acid | |
---|---|---|
Source | PubChem | |
URL | https://pubchem.ncbi.nlm.nih.gov | |
Description | Data deposited in or computed by PubChem | |
InChI |
InChI=1S/C3H8N2O2/c4-1-2(5)3(6)7/h2H,1,4-5H2,(H,6,7)/t2-/m0/s1 | |
Source | PubChem | |
URL | https://pubchem.ncbi.nlm.nih.gov | |
Description | Data deposited in or computed by PubChem | |
InChI Key |
PECYZEOJVXMISF-REOHCLBHSA-N | |
Source | PubChem | |
URL | https://pubchem.ncbi.nlm.nih.gov | |
Description | Data deposited in or computed by PubChem | |
Canonical SMILES |
C(C(C(=O)O)N)N | |
Source | PubChem | |
URL | https://pubchem.ncbi.nlm.nih.gov | |
Description | Data deposited in or computed by PubChem | |
Isomeric SMILES |
C([C@@H](C(=O)O)N)N | |
Source | PubChem | |
URL | https://pubchem.ncbi.nlm.nih.gov | |
Description | Data deposited in or computed by PubChem | |
Molecular Formula |
C3H8N2O2 | |
Source | PubChem | |
URL | https://pubchem.ncbi.nlm.nih.gov | |
Description | Data deposited in or computed by PubChem | |
DSSTOX Substance ID |
DTXSID80193328 | |
Record name | L-Alanine, 3-amino- | |
Source | EPA DSSTox | |
URL | https://comptox.epa.gov/dashboard/DTXSID80193328 | |
Description | DSSTox provides a high quality public chemistry resource for supporting improved predictive toxicology. | |
Molecular Weight |
104.11 g/mol | |
Source | PubChem | |
URL | https://pubchem.ncbi.nlm.nih.gov | |
Description | Data deposited in or computed by PubChem | |
Physical Description |
Solid | |
Record name | 2,3-Diaminopropionic acid | |
Source | Human Metabolome Database (HMDB) | |
URL | http://www.hmdb.ca/metabolites/HMDB0002006 | |
Description | The Human Metabolome Database (HMDB) is a freely available electronic database containing detailed information about small molecule metabolites found in the human body. | |
Explanation | HMDB is offered to the public as a freely available resource. Use and re-distribution of the data, in whole or in part, for commercial purposes requires explicit permission of the authors and explicit acknowledgment of the source material (HMDB) and the original publication (see the HMDB citing page). We ask that users who download significant portions of the database cite the HMDB paper in any resulting publications. | |
CAS No. |
4033-39-0 | |
Record name | L-α,β-Diaminopropionic acid | |
Source | CAS Common Chemistry | |
URL | https://commonchemistry.cas.org/detail?cas_rn=4033-39-0 | |
Description | CAS Common Chemistry is an open community resource for accessing chemical information. Nearly 500,000 chemical substances from CAS REGISTRY cover areas of community interest, including common and frequently regulated chemicals, and those relevant to high school and undergraduate chemistry classes. This chemical information, curated by our expert scientists, is provided in alignment with our mission as a division of the American Chemical Society. | |
Explanation | The data from CAS Common Chemistry is provided under a CC-BY-NC 4.0 license, unless otherwise stated. | |
Record name | 2,3-Diaminopropionic acid, (+)- | |
Source | ChemIDplus | |
URL | https://pubchem.ncbi.nlm.nih.gov/substance/?source=chemidplus&sourceid=0004033390 | |
Description | ChemIDplus is a free, web search system that provides access to the structure and nomenclature authority files used for the identification of chemical substances cited in National Library of Medicine (NLM) databases, including the TOXNET system. | |
Record name | L-Alanine, 3-amino- | |
Source | EPA DSSTox | |
URL | https://comptox.epa.gov/dashboard/DTXSID80193328 | |
Description | DSSTox provides a high quality public chemistry resource for supporting improved predictive toxicology. | |
Record name | 2,3-DIAMINOPROPIONIC ACID, (+)- | |
Source | FDA Global Substance Registration System (GSRS) | |
URL | https://gsrs.ncats.nih.gov/ginas/app/beta/substances/JE1TUV83JA | |
Description | The FDA Global Substance Registration System (GSRS) enables the efficient and accurate exchange of information on what substances are in regulated products. Instead of relying on names, which vary across regulatory domains, countries, and regions, the GSRS knowledge base makes it possible for substances to be defined by standardized, scientific descriptions. | |
Explanation | Unless otherwise noted, the contents of the FDA website (www.fda.gov), both text and graphics, are not copyrighted. They are in the public domain and may be republished, reprinted and otherwise used freely by anyone without the need to obtain permission from FDA. Credit to the U.S. Food and Drug Administration as the source is appreciated but not required. | |
Record name | 2,3-Diaminopropionic acid | |
Source | Human Metabolome Database (HMDB) | |
URL | http://www.hmdb.ca/metabolites/HMDB0002006 | |
Description | The Human Metabolome Database (HMDB) is a freely available electronic database containing detailed information about small molecule metabolites found in the human body. | |
Explanation | HMDB is offered to the public as a freely available resource. Use and re-distribution of the data, in whole or in part, for commercial purposes requires explicit permission of the authors and explicit acknowledgment of the source material (HMDB) and the original publication (see the HMDB citing page). We ask that users who download significant portions of the database cite the HMDB paper in any resulting publications. | |
Retrosynthesis Analysis
AI-Powered Synthesis Planning: Our tool employs the Template_relevance Pistachio, Template_relevance Bkms_metabolic, Template_relevance Pistachio_ringbreaker, Template_relevance Reaxys, Template_relevance Reaxys_biocatalysis model, leveraging a vast database of chemical reactions to predict feasible synthetic routes.
One-Step Synthesis Focus: Specifically designed for one-step synthesis, it provides concise and direct routes for your target compounds, streamlining the synthesis process.
Accurate Predictions: Utilizing the extensive PISTACHIO, BKMS_METABOLIC, PISTACHIO_RINGBREAKER, REAXYS, REAXYS_BIOCATALYSIS database, our tool offers high-accuracy predictions, reflecting the latest in chemical research and data.
Strategy Settings
Precursor scoring | Relevance Heuristic |
---|---|
Min. plausibility | 0.01 |
Model | Template_relevance |
Template Set | Pistachio/Bkms_metabolic/Pistachio_ringbreaker/Reaxys/Reaxys_biocatalysis |
Top-N result to add to graph | 6 |
Feasible Synthetic Routes
Disclaimer and Information on In-Vitro Research Products
Please be aware that all articles and product information presented on BenchChem are intended solely for informational purposes. The products available for purchase on BenchChem are specifically designed for in-vitro studies, which are conducted outside of living organisms. In-vitro studies, derived from the Latin term "in glass," involve experiments performed in controlled laboratory settings using cells or tissues. It is important to note that these products are not categorized as medicines or drugs, and they have not received approval from the FDA for the prevention, treatment, or cure of any medical condition, ailment, or disease. We must emphasize that any form of bodily introduction of these products into humans or animals is strictly prohibited by law. It is essential to adhere to these guidelines to ensure compliance with legal and ethical standards in research and experimentation.