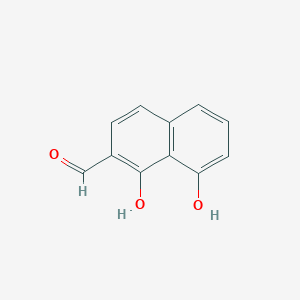
1,8-Dihydroxy-2-naphthaldehyde
Overview
Description
1,8-Dihydroxy-2-naphthaldehyde (DHNA) is a naphthaldehyde derivative featuring two hydroxyl groups at positions 1 and 8 and an aldehyde group at position 2. This unique arrangement enables the formation of two intramolecular hydrogen bonds (IHBs) between the hydroxyl and aldehyde groups, making DHNA a prototypical system for studying excited-state double proton transfer (ESDPT) .
Key photophysical properties include:
- Stepwise ESDPT mechanism: The first proton transfer occurs ultrafast (<150 fs) and irreversibly, while the second is reversible with forward/backward rates of ~1.7 ps⁻¹ and 3.6 ps⁻¹, respectively .
- Large Stokes shift: Emission peaks shift from 602 nm to 513 nm under external electric fields (EEFs), demonstrating tunable photophysics .
- Theoretical validation: Density functional theory (DFT) and multistate complete active space (MS-CASPT2) studies confirm the stepwise pathway and energy barriers .
Mechanism of Action
Target of Action
The primary target of 1,8-Dihydroxy-2-naphthaldehyde is the excited state double proton transfer (ESDPT) process . This process involves the transfer of protons in the excited state, which is a crucial aspect of many biological and chemical reactions .
Mode of Action
This compound interacts with its targets through a stepwise ESDPT mechanism . Specifically, the first proton transfer from S1-ENOL to S1-KETO-1 is barrierless, whereas the second one from S1-KETO-1 to S1-KETO-2 demands a barrier of approximately 6.0 kcal/mol . This stepwise mechanism is crucial for the compound’s interaction with its targets .
Biochemical Pathways
The ESDPT process of this compound affects the potential energy surfaces (PESs), leading to changes in the energy barrier . Increasing the positive external electric field (EEF) results in a decrease in the energy barrier, while vice versa . These changes in the PESs and energy barrier can have significant downstream effects on various biochemical pathways .
Pharmacokinetics
Its mode of action suggests that its bioavailability could be influenced by factors such as the presence of eefs .
Result of Action
The result of this compound’s action is the occurrence of dual-emission bands . This is due to the trapping of the compound in the S1 state, which enables these dual-emission bands . This property makes this compound potentially useful for designing novel molecular materials with excellent photoluminescent performances .
Action Environment
The action of this compound is influenced by environmental factors, particularly EEFs . The ESDPT process of the compound can be affected by distinct EEFs . For instance, when the EEF changes from +10 × 10 −4 a.u. to +20 × 10 −4 a.u., the fluorescence peak undergoes a blue shift from 602 nm to 513 nm in the keto2 form . This suggests that the compound’s action, efficacy, and stability can be regulated and controlled by manipulating the EEF .
Biochemical Analysis
Biochemical Properties
1,8-Dihydroxy-2-naphthaldehyde plays a significant role in biochemical reactions, particularly in the ESDPT process . The ESDPT process of this compound is affected by distinct external electric fields (EEFs) . The intramolecular hydrogen bond (IHB) parameters, including bond lengths and angles, change in the distinct EEFs .
Molecular Mechanism
The molecular mechanism of this compound involves the ESDPT process . The potential energy surfaces (PESs) indicate that the ESDPT process of this compound is stepwise . Increasing the positive EEF results in a decrease in the energy barrier accordingly, while vice versa .
Temporal Effects in Laboratory Settings
In laboratory settings, the effects of this compound can change over time. For instance, the absorption and fluorescence spectra undergo a corresponding red or blue shift in the EEF
Biological Activity
1,8-Dihydroxy-2-naphthaldehyde (DHNA), a naphthalene derivative characterized by two hydroxyl groups and an aldehyde group, has garnered attention for its significant biological activities. This article explores the compound's chemical properties, biological effects, and potential applications based on diverse research findings.
The structure of DHNA enhances its reactivity due to the presence of both hydroxy and aldehyde functional groups. The hydroxy groups improve solubility and provide sites for further functionalization, while the aldehyde group facilitates condensation reactions crucial for synthesizing complex organic molecules .
Biological Activities
-
Antibacterial and Antifungal Activity
- Studies have shown that DHNA exhibits moderate to significant antibacterial activity against various bacterial strains, including Staphylococcus aureus and Escherichia coli. Additionally, it demonstrates good antifungal activity against several fungal species .
- A study involving metal complexes of DHNA indicated enhanced antibacterial properties compared to the free ligand, suggesting that metal coordination amplifies its biological efficacy .
-
Cytotoxicity
- The cytotoxic effects of DHNA have been assessed using the brine shrimp bioassay, revealing notable cytotoxic properties against Artemia salina . Furthermore, metal complexes derived from DHNA were evaluated for their anticancer activities against human cancer cell lines such as HepG2 (hepatocellular carcinoma) and HCT-116 (colon carcinoma), showing promising results .
-
Excited-State Proton Transfer
- DHNA is notable for its photophysical properties, particularly in excited-state intramolecular double proton transfer (ESIDPT). Research indicates that upon excitation, DHNA undergoes rapid proton transfer processes that are ultrafast (<150 fs) and reversible under certain conditions . This property is significant for applications in fluorescence labeling and sensing.
Research Findings
Case Studies
- Antibacterial Efficacy
- Photophysical Properties
Scientific Research Applications
Structural Characteristics and Synthesis
DHNA is characterized by two hydroxyl groups at the 1 and 8 positions of the naphthalene ring, which facilitate intramolecular hydrogen bonding. The compound is synthesized through a multi-step process involving the reaction of naphthalene-1,8-diol with various reagents to introduce the aldehyde group effectively. The synthesis has been documented to yield DHNA with high purity, confirmed through X-ray crystallography and NMR spectroscopy .
Excited-State Intramolecular Double Proton Transfer (ESIDPT)
One of the most notable applications of DHNA is its role in studying excited-state intramolecular double proton transfer (ESIDPT). Research has shown that DHNA undergoes a stepwise double-proton transfer upon photoexcitation, which is crucial for understanding fundamental chemical processes. The first proton transfer occurs ultrafast (within 150 fs), while the second is reversible with rates around 1.7 ps for forward and 3.6 ps for backward transfers .
Mechanistic Insights
The mechanistic insights gained from studying DHNA's ESIDPT behavior contribute significantly to the broader understanding of proton relay systems in biological and chemical contexts. Theoretical models utilizing density functional theory (DFT) and time-dependent DFT (TDDFT) have elucidated the dynamics of these processes, providing a framework for future studies on similar compounds .
Photophysical Properties
DHNA exhibits intriguing photophysical properties, including dual emission bands upon excitation. The absorption spectrum shows a maximum at approximately 400 nm, attributed to π-π* transitions, while emission bands are observed at 520 nm and 650 nm corresponding to different excited states (TA* and TB*). These characteristics make DHNA a valuable model for investigating excited-state behaviors in related compounds .
Experimental Studies on DHNA
Several studies have been conducted to analyze the excited-state dynamics of DHNA:
- Peng et al. (2015) : This study demonstrated the stepwise double-proton transfer mechanism using time-resolved fluorescence spectroscopy, providing experimental evidence for the theoretical predictions regarding the ultrafast nature of the first proton transfer .
- Further Investigations : Subsequent research has focused on modifying the substituents on the naphthalene ring to explore how these changes affect proton transfer efficiencies and photophysical properties, thus expanding the applicability of DHNA in various chemical contexts .
Material Science
Due to its unique properties, DHNA can be explored as a potential candidate for optoelectronic materials, where its ability to undergo rapid proton transfers may contribute to enhanced performance in devices such as sensors or light-emitting diodes.
Biological Systems
The insights gained from studying DHNA's proton transfer mechanisms may also have implications in biological systems where similar processes occur, such as enzyme catalysis and energy transfer in photosynthesis.
Chemical Reactions Analysis
Excited-State Double Proton Transfer (ESDPT)
DHNA undergoes a stepwise ESDPT mechanism upon photoexcitation (λ<sub>ex</sub> = 400 nm), as demonstrated by femtosecond fluorescence upconversion and DFT calculations :
Key Findings:
-
First Proton Transfer (N* → TA*) :
-
Second Proton Transfer (TA* ↔ TB*) :
Table 1: ESDPT Kinetics and Emission Properties
Reversible Proton Relay in the Excited State
The second proton transfer follows a relay mechanism (TA* ↔ TB*), forming a thermodynamic equilibrium supported by potential energy surface calculations (Figure 7 in ). Electron density difference (EDD) maps reveal charge redistribution favoring proton transfer post-excitation .
Condensation and Functionalization Reactions
The aldehyde group in DHNA enables condensation reactions, making it valuable for synthesizing:
Structural Advantages :
-
Dual hydroxyl groups enhance solubility and provide sites for further functionalization (e.g., metal coordination) .
-
Extended π-conjugation supports applications in optoelectronic materials .
Theoretical Insights
DFT calculations (B3LYP/6-31G*) corroborate the stepwise ESDPT pathway, showing:
Q & A
Basic Research Questions
Q. What is the mechanism of excited-state intramolecular double proton transfer (ESIDPT) in 1,8-dihydroxy-2-naphthaldehyde, and how is it experimentally characterized?
The ESIDPT process occurs in a stepwise manner: the first proton transfer (O1–H→O2) is ultrafast (<150 fs) and irreversible, while the second (O8–H→O2) is reversible, with forward/backward rates of ~1.7 ps⁻¹ and ~3.6 ps⁻¹, respectively . Experimental characterization involves time-resolved fluorescence spectroscopy, where fluorescence decay kinetics are analyzed using a three-species model (normal form, tautomer 1, tautomer 2) to account for population dynamics . Ultrafast laser systems with sub-picosecond resolution are critical for resolving the first step .
Q. How do adjacent hydroxyl groups at positions 1 and 8 influence the ESIDPT process?
The proximity of the hydroxyl groups enables a relay mechanism for sequential proton transfer. The first proton transfer creates a keto intermediate, which facilitates the second transfer via hydrogen-bond rearrangement. Structural analysis using DFT (B3LYP/6-31+G(d,p)) confirms that the intramolecular hydrogen-bond network stabilizes the tautomeric forms . Adjacent hydroxyl groups are essential for enabling the stepwise pathway, as single hydroxyl analogs (e.g., 2-naphthaldehyde) exhibit only single proton transfer .
Q. What experimental models are used to analyze fluorescence decay kinetics in stepwise ESIDPT systems?
A three-species kinetic model is applied, incorporating rate equations for the normal form (N*), first tautomer (T1*), and second tautomer (T2*). This model accounts for the near-instantaneous depletion of N* after excitation and the reversible equilibrium between T1* and T2*. Global fitting of time-resolved fluorescence data at multiple emission wavelengths validates the model . Challenges arise when the first proton transfer is faster than the instrument response (~150 fs), requiring assumptions about initial conditions .
Advanced Research Questions
Q. How do computational methods (TD-DFT vs. MS-CASPT2) differ in modeling ESIDPT dynamics, and what are their limitations?
- TD-DFT (B3LYP/6-31+G(d,p)) : Efficient for ground-state geometry optimization but may underestimate excited-state barriers due to single-configuration approximations .
- MS-CASPT2//CASSCF : Multi-configurational approach captures static correlation and conical intersections, critical for accurately modeling excited-state potential energy surfaces. This method predicts the irreversible first transfer and reversible second transfer, aligning with experimental rates . Discrepancies arise in predicting tautomer stability, highlighting the need for hybrid experimental-theoretical validation .
Q. What methodological challenges exist in resolving ultrafast proton transfer kinetics, and how are they addressed?
The first ESIPT step (<150 fs) exceeds the temporal resolution of conventional streak cameras, necessitating indirect methods:
- Femtosecond transient absorption : Probes spectral shifts associated with tautomer formation .
- Fluorescence upconversion : Achieves ~100 fs resolution to track initial depletion of the normal form .
- Kinetic modeling : Assumes the first step is instantaneous, focusing on fitting the reversible second step . Cross-validation with computational data (e.g., MS-CASPT2) ensures consistency .
Q. How can discrepancies between theoretical predictions and experimental proton transfer rates be resolved?
Discrepancies often stem from solvent effects, vibrational relaxation, or approximations in computational methods. Strategies include:
- Explicit solvent modeling : Incorporating polarizable continuum models (PCM) or molecular dynamics to account for solvent interactions .
- Vibronic coupling analysis : Evaluating how low-frequency modes (e.g., hydrogen-bond stretching) modulate reaction barriers .
- Experimental-theoretical synergy : Using temperature-dependent fluorescence data to refine computed activation energies .
Q. Data Contradiction Analysis
Q. Why do some studies report a two-species model for ESIDPT, while others require three species?
Early studies assumed a two-species model (N* and T2*) due to the rapid depletion of N*, but this fails to capture the reversible T1↔T2 equilibrium. The three-species model resolves this by including T1*, validated via global fitting of multi-wavelength decay curves . Contradictions arise from instrumentation limitations: slower detection systems mask T1* dynamics, leading to oversimplified models .
Q. Methodological Tables
Table 1: Key Experimental and Computational Parameters for ESIDPT
Table 2: Advantages and Limitations of Computational Methods
Method | Advantages | Limitations |
---|---|---|
TD-DFT | Fast, suitable for ground-state geometry | Underestimates excited-state barriers |
MS-CASPT2 | Accurate for multi-configurational states | Computationally expensive |
CASSCF | Captures conical intersections | Requires expert parameterization |
Comparison with Similar Compounds
Comparison with Structurally Similar Compounds
1-Hydroxy-2-naphthaldehyde (HN12)
- Structure : Single hydroxyl group at position 1 and aldehyde at position 2.
- Proton Transfer : Single excited-state intramolecular proton transfer (ESIPT) with a six-membered transition state .
- Key Differences :
1,8-Dihydroxynaphthalene
- Proton Transfer: No ESIPT or ESDPT observed due to absence of IHB-stabilizing aldehyde .
- Key Differences :
- Demonstrates how the aldehyde group in DHNA is critical for IHB formation and proton transfer.
1,2-Dihydroxyanthraquinone
- Structure: Adjacent hydroxyl groups on an anthraquinone backbone.
- Proton Transfer : Single ESIPT with a six-membered transition state, similar to HN12 .
- Key Differences: Slower proton transfer kinetics (picosecond timescale) compared to DHNA’s femtosecond first step . Anthraquinone’s extended π-system alters absorption/emission profiles.
3,6-Dihydroxy-4,5-dimethylbenzene-1,2-dicarbaldehyde
- Structure : Two aldehyde groups and hydroxyls on a benzene ring.
- Proton Transfer: No reported ESIPT/ESDPT despite IHBs, likely due to steric hindrance from methyl groups .
- Key Differences :
- Highlights the necessity of adjacent hydroxyl-aldehyde pairs for efficient proton transfer.
Comparative Data Table
Compound | ESIPT/ESDPT Type | Proton Transfer Rates | Stokes Shift (nm) | Key Functional Groups |
---|---|---|---|---|
DHNA | Stepwise ESDPT | 1st: <150 fs; 2nd: 1.7/3.6 ps⁻¹ | 250–350 | 1,8-OH; 2-CHO |
HN12 | Single ESIPT | ~1 ps⁻¹ | ~150 | 1-OH; 2-CHO |
1,8-Dihydroxynaphthalene | None | N/A | N/A | 1,8-OH; no CHO |
1,2-Dihydroxyanthraquinone | Single ESIPT | ~2–10 ps⁻¹ | ~180 | Adjacent OH on anthraquinone |
3,6-Dihydroxy-...-dicarbaldehyde | None | N/A | N/A | 3,6-OH; 1,2-CHO; methyl groups |
Mechanistic Insights from Theoretical Studies
- DHNA : TDDFT and MS-CASPT2 calculations reveal a relay mechanism where the first proton transfer lowers the energy barrier for the second . External electric fields (EEFs) modulate IHB strength, enabling spectral tuning .
- Contrast with Anthraquinone: Anthraquinone’s proton transfer involves a single transition state, lacking the asymmetry that drives DHNA’s stepwise process .
Preparation Methods
Synthetic Route Overview
The synthesis of DHNA typically involves the following key steps:
- Starting Material: Naphthalene-1,8-diol (commercially available)
- Protection of Hydroxyl Groups: Conversion of hydroxyl groups to methoxymethyl ethers
- Formylation: Introduction of the aldehyde group at the 2-position
- Deprotection: Removal of protecting groups to regenerate the dihydroxy functionalities
This sequence ensures selective functionalization and preserves the integrity of the sensitive hydroxyl groups during the formylation step.
Detailed Stepwise Preparation
Step No. | Reaction Description | Reagents and Conditions | Outcome/Notes |
---|---|---|---|
1 | Protection of hydroxyl groups | Naphthalene-1,8-diol reacted with NaH and chloromethyl methyl ether | Formation of 1,8-bis(methoxymethoxy)naphthalene (compound 2) with protected hydroxyls to prevent side reactions |
2 | Formylation at 2-position | Treatment of compound 2 with n-butyllithium (n-BuLi) and TMEDA, followed by DMF addition | Introduction of aldehyde group yielding 1,8-bis(methoxymethoxy)-2-naphthaldehyde (compound 3) |
3 | Deprotection of methoxymethyl groups | Acidic conditions applied to compound 3 | Regeneration of free hydroxyl groups, producing 1,8-dihydroxy-2-naphthaldehyde (DHNA) |
This synthetic strategy was reported with good overall yields and confirmed by X-ray crystallography, validating the structure and purity of the final product.
Experimental Findings and Characterization
- Structural Confirmation: Single-crystal X-ray diffraction analysis confirmed the molecular structure of DHNA, showing the presence of two intramolecular hydrogen bonds between the hydroxyl groups and the aldehyde oxygen.
- Spectroscopic Evidence: The compound exhibits characteristic absorption and emission spectra due to its unique intramolecular proton transfer properties, which are directly related to its structural features obtained from the synthetic route.
Research Insights on Synthesis
- The protection-deprotection strategy is crucial to avoid side reactions during the lithiation and formylation steps.
- The use of n-BuLi and TMEDA facilitates selective lithiation at the 2-position, enabling efficient formylation with DMF.
- Acidic deprotection conditions are optimized to remove methoxymethyl groups without degrading the aldehyde or hydroxyl functionalities.
- The synthetic approach allows for the preparation of DHNA in sufficient quantities for detailed photophysical and proton transfer studies.
Summary Table of Preparation Methods
Aspect | Details |
---|---|
Starting Material | Naphthalene-1,8-diol |
Protection Reagents | Sodium hydride (NaH), chloromethyl methyl ether |
Formylation Reagents | n-Butyllithium (n-BuLi), TMEDA, DMF |
Deprotection Conditions | Acidic medium (specific acids not detailed in source, typically mild acid conditions) |
Key Intermediate Compounds | 1,8-bis(methoxymethoxy)naphthalene (2), 1,8-bis(methoxymethoxy)-2-naphthaldehyde (3) |
Characterization Techniques | X-ray crystallography, IR spectroscopy, UV-Vis absorption, fluorescence spectroscopy |
Yield and Purity | Good yields reported; purity confirmed by crystallography and spectroscopic methods |
Properties
IUPAC Name |
1,8-dihydroxynaphthalene-2-carbaldehyde | |
---|---|---|
Source | PubChem | |
URL | https://pubchem.ncbi.nlm.nih.gov | |
Description | Data deposited in or computed by PubChem | |
InChI |
InChI=1S/C11H8O3/c12-6-8-5-4-7-2-1-3-9(13)10(7)11(8)14/h1-6,13-14H | |
Source | PubChem | |
URL | https://pubchem.ncbi.nlm.nih.gov | |
Description | Data deposited in or computed by PubChem | |
InChI Key |
QBXCVGGCXRBENM-UHFFFAOYSA-N | |
Source | PubChem | |
URL | https://pubchem.ncbi.nlm.nih.gov | |
Description | Data deposited in or computed by PubChem | |
Canonical SMILES |
C1=CC2=C(C(=C1)O)C(=C(C=C2)C=O)O | |
Source | PubChem | |
URL | https://pubchem.ncbi.nlm.nih.gov | |
Description | Data deposited in or computed by PubChem | |
Molecular Formula |
C11H8O3 | |
Source | PubChem | |
URL | https://pubchem.ncbi.nlm.nih.gov | |
Description | Data deposited in or computed by PubChem | |
DSSTOX Substance ID |
DTXSID60826869 | |
Record name | 1,8-Dihydroxynaphthalene-2-carbaldehyde | |
Source | EPA DSSTox | |
URL | https://comptox.epa.gov/dashboard/DTXSID60826869 | |
Description | DSSTox provides a high quality public chemistry resource for supporting improved predictive toxicology. | |
Molecular Weight |
188.18 g/mol | |
Source | PubChem | |
URL | https://pubchem.ncbi.nlm.nih.gov | |
Description | Data deposited in or computed by PubChem | |
CAS No. |
858457-19-9 | |
Record name | 1,8-Dihydroxynaphthalene-2-carbaldehyde | |
Source | EPA DSSTox | |
URL | https://comptox.epa.gov/dashboard/DTXSID60826869 | |
Description | DSSTox provides a high quality public chemistry resource for supporting improved predictive toxicology. | |
Retrosynthesis Analysis
AI-Powered Synthesis Planning: Our tool employs the Template_relevance Pistachio, Template_relevance Bkms_metabolic, Template_relevance Pistachio_ringbreaker, Template_relevance Reaxys, Template_relevance Reaxys_biocatalysis model, leveraging a vast database of chemical reactions to predict feasible synthetic routes.
One-Step Synthesis Focus: Specifically designed for one-step synthesis, it provides concise and direct routes for your target compounds, streamlining the synthesis process.
Accurate Predictions: Utilizing the extensive PISTACHIO, BKMS_METABOLIC, PISTACHIO_RINGBREAKER, REAXYS, REAXYS_BIOCATALYSIS database, our tool offers high-accuracy predictions, reflecting the latest in chemical research and data.
Strategy Settings
Precursor scoring | Relevance Heuristic |
---|---|
Min. plausibility | 0.01 |
Model | Template_relevance |
Template Set | Pistachio/Bkms_metabolic/Pistachio_ringbreaker/Reaxys/Reaxys_biocatalysis |
Top-N result to add to graph | 6 |
Feasible Synthetic Routes
Disclaimer and Information on In-Vitro Research Products
Please be aware that all articles and product information presented on BenchChem are intended solely for informational purposes. The products available for purchase on BenchChem are specifically designed for in-vitro studies, which are conducted outside of living organisms. In-vitro studies, derived from the Latin term "in glass," involve experiments performed in controlled laboratory settings using cells or tissues. It is important to note that these products are not categorized as medicines or drugs, and they have not received approval from the FDA for the prevention, treatment, or cure of any medical condition, ailment, or disease. We must emphasize that any form of bodily introduction of these products into humans or animals is strictly prohibited by law. It is essential to adhere to these guidelines to ensure compliance with legal and ethical standards in research and experimentation.