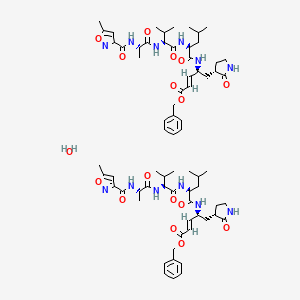
Mpro inhibitor N3 hemihydrate
- Click on QUICK INQUIRY to receive a quote from our team of experts.
- With the quality product at a COMPETITIVE price, you can focus more on your research.
Overview
Description
Mpro inhibitor N3 hemihydrate is a mechanism-based, peptidomimetic inhibitor designed to target the main protease (Mpro) of coronaviruses, including SARS-CoV-2. Mpro, a chymotrypsin-like protease, is essential for viral replication by cleaving polyproteins into functional non-structural proteins. N3 binds covalently to the catalytic cysteine (Cys145) of Mpro, forming a stable complex that inhibits protease activity .
Preparation Methods
The synthesis of Mpro inhibitor N3 hemihydrate involves the use of peptidyl Michael acceptors. The preparation typically follows a two-step mechanism, involving the formation of a covalent enzyme-inhibitor product . The synthetic routes and reaction conditions are designed to ensure the formation of the desired inhibitor with high specificity and potency . Industrial production methods would likely involve large-scale synthesis using optimized reaction conditions to maximize yield and purity.
Chemical Reactions Analysis
Covalent Bond Formation with Catalytic Cysteine
N3 inhibits Mpro through a Michael addition reaction between its α,β-unsaturated ester warhead and the thiol group of Cys145 in the enzyme's active site . Key reaction steps include:
-
Nucleophilic attack : The deprotonated sulfur atom (Sγ) of Cys145 attacks the β-carbon of N3's vinyl group, forming a covalent C-S bond (1.8 Å bond length observed crystallographically) .
-
Proton transfer : His41 acts as a general base, facilitating proton transfer from Cys145 to the α-carbon of N3 .
Critical structural features :
-
The reaction proceeds via a CysS⁻/HisH⁺ ion pair transition state, stabilized by hydrogen bonds with backbone atoms of Gly143 and Ser144 .
-
X-ray crystallography (PDB: 6LU7) confirms irreversible covalent adduct formation .
Michael Addition Mechanism
QM/MM simulations reveal a concerted asynchronous mechanism with the following parameters :
Reaction Stage | Activation Energy (kcal/mol) | Key Bond Lengths (Å) |
---|---|---|
Cys145 attack on β-carbon (TS1) | 11.2 | Cβ-Sγ: 2.14 |
Proton transfer (TS2) | 9.8 | O-Hδ: 1.39 |
-
The reaction is exergonic (ΔG = -17.9 kcal/mol), favoring irreversible inhibition .
-
Comparative studies with derivatives B1 and B2 show lower activation barriers (9.8 kcal/mol for B2), suggesting tunable reactivity .
Kinetic Parameters of Inhibition
Time-dependent kinetics characterize N3's interaction with Mpro :
Parameter | Value | Method |
---|---|---|
k<sub>inact</sub>/K<sub>i</sub> | 1.4 × 10⁴ M⁻¹s⁻¹ | Pre-steady-state kinetics |
Half-life of inhibition | < 5 minutes | Stopped-flow assay |
-
Inhibition follows a two-step mechanism :
Structural Determinants of Reactivity
N3's chemical reactivity is modulated by specific interactions in Mpro's substrate-binding pockets :
Subsite Interactions :
Subsite | Residues Involved | Interaction Type |
---|---|---|
S1 | H163, E166, F140 | Hydrogen bonding |
S2 | M49, Y54, M165 | Hydrophobic packing |
S1' | T24, T25 | Van der Waals contacts |
-
The lactam group at P1 forms critical hydrogen bonds with H163 and backbone atoms .
-
Hydrophobic residues at P2 (Leu) enhance binding affinity through S2 subsite interactions.
Stability and Selectivity Considerations
Scientific Research Applications
Mpro inhibitor N3 hemihydrate has a wide range of scientific research applications, including:
Chemistry: Used as a biochemical probe to study the inhibition of viral proteases.
Biology: Helps in understanding the molecular mechanisms of viral replication and inhibition.
Medicine: Potential therapeutic agent for treating COVID-19 and other coronavirus-related diseases
Industry: Used in the development of antiviral drugs and treatments.
Mechanism of Action
The mechanism of action of Mpro inhibitor N3 hemihydrate involves the inhibition of the main protease (Mpro) of SARS-CoV-2. The compound forms a covalent bond with the protease, preventing it from processing viral polyproteins necessary for viral replication . This inhibition effectively halts the replication of the virus, making it a promising candidate for antiviral therapy .
Comparison with Similar Compounds
Key Properties
- Broad-spectrum activity : Inhibits Mpro from SARS-CoV-2 (IC₅₀ = 16.77 μM), SARS-CoV, MERS-CoV, HCoV-229E, and feline infectious peritonitis virus (FIPV) with IC₅₀ values ranging from 2.7 to 8.8 μM .
- Structural basis : Co-crystallized with SARS-CoV-2 Mpro (PDB: 6LU7), revealing interactions with catalytic dyad (Cys145-His41) and substrate-binding residues (Glu166, Gln189) .
- Antiviral efficacy : Demonstrates cell permeability and inhibits viral replication in vitro (EC₅₀ = 0.64 μM for IBV virus) .
Natural Compounds
Several natural compounds exhibit superior binding affinity and interaction profiles compared to N3 (Table 1).
Table 1: Natural Compound Comparison
Notes:
- ZINC000003947429 and ZINC000003594862 exhibit higher binding affinities than N3, with additional interactions at conserved residues (e.g., His41) critical for catalytic activity .
- Withanone and CAPE (caffeic acid phenethyl ester) show binding energies comparable to N3 but require validation in cellular assays .
Repurposed Drugs and Database Screens
DrugBank Compounds :
- CHEMBL562054 : Binds Gly143, Ser144, and Cys145 with a docking score of -9.5 kcal/mol, surpassing N3 (-7.3 kcal/mol) .
- DB07458 : A protein kinase C inhibitor with dual indole rings, showing strong interactions with Mpro’s catalytic pocket .
QSAR Models :
- A pharmacophore model identified 48 Mpro inhibitors, with natural compounds (e.g., podocarpus flavon-B) showing micromolar activity against SARS-CoV Mpro .
Binding Free Energy and Stability
MM/GBSA Analysis :
Compound | ΔG Binding (kcal/mol) | Key Energy Contributors |
---|---|---|
N3 inhibitor | -45.2 | Coulomb (-32.1), vdW (-13.1) |
ZINC000043552589 | -58.7 | Coulomb (-40.3), vdW (-18.4) |
ZINC000003947429 | -62.1 | Coulomb (-43.8), vdW (-18.3) |
Notes:
- Natural compounds exhibit stronger Coulomb and van der Waals contributions, stabilizing Mpro complexes more effectively than N3 .
Q & A
Basic Research Questions
Q. What is the molecular mechanism by which N3 hemihydrate inhibits Mpro, and how is its covalent binding characterized?
N3 hemihydrate acts as a covalent inhibitor of Mpro via a Michael addition reaction, forming a stable bond with the catalytic cysteine residue (Cys145) in the protease's active site. This interaction disrupts Mpro's proteolytic function, critical for viral replication. Structural studies (PDB ID: 6LU7) confirm that N3 occupies the substrate-binding pocket, forming hydrogen bonds with residues N142, G143, E166, and Q189, and a salt bridge with E166, which stabilizes the dimerization interface .
Q. How does X-ray crystallography validate the binding pose and interactions of N3 with Mpro?
The co-crystal structure of Mpro-N3 (6LU7) reveals that N3 adopts a conformation mimicking the natural substrate, with its warhead oriented toward Cys145. The asymmetric unit shows precise positioning of the inhibitor's P1-P3 groups, critical for mimicking polyprotein cleavage sites. This structural data guides mutagenesis studies and pharmacophore modeling .
Q. What in vitro efficacy data support N3 hemihydrate as a broad-spectrum coronavirus inhibitor?
N3 exhibits inhibitory activity against multiple coronaviruses, including SARS-CoV-2 (EC50: 16.77 µM), SARS-CoV, MERS-CoV, HCoV-229E (IC50: 4.0 µM), and feline infectious peritonitis virus (FIPV; IC50: 8.8 µM). Its ability to penetrate cells and block viral replication at early infection stages (e.g., in IBV embryos) highlights its broad-spectrum potential .
Advanced Research Questions
Q. How do QM/MM simulations elucidate the chemical reactivity and thermodynamic stability of N3-Mpro complexes?
Quantum mechanics/molecular mechanics (QM/MM) studies reveal that N3's inhibition is exothermic (ΔG = -17.9 kcal/mol), with a low alkylation barrier due to optimal alignment of its Michael acceptor warhead and catalytic dyad (Cys145-His41). Modifications to the recognition moiety (e.g., in derivatives B1/B2) alter reaction energetics, enabling tunable reversibility .
Q. What methodological considerations are critical when analyzing binding free energy across molecular dynamics (MD) frames?
MD simulations show that N3's binding stability varies across conformational frames. For robust analysis, researchers should:
- Use MM-GBSA to calculate ensemble-averaged binding free energy.
- Prioritize frames where N3's interactions (e.g., with H41, E166) align with crystallographic data.
- Exclude transient conformations with poor hydrogen-bond occupancy (<50%) .
Q. How can virtual screening optimize N3 derivatives for improved binding affinity and selectivity?
Structure-based virtual screening leverages the 6LU7 template to:
- Identify compounds with complementary interactions to Mpro's S1-S4 subsites.
- Filter candidates using g-score thresholds (e.g., < -8 kcal/mol) and ADMET properties.
- Validate hits via cross-docking against multiple Mpro conformations to avoid false positives .
Q. What experimental approaches validate synergistic inhibition between N3 and metal ions like Zn²+?
Biochemical assays demonstrate that Zn²+ (a non-competitive inhibitor) enhances N3's irreversible inhibition of FIPV Mpro. Synergy is validated via:
- Co-crystallization showing dual occupancy in the active site.
- Dose-response matrices (e.g., Chou-Talalay plots) quantifying combination index (CI) values .
Q. How do structural fluctuations in Mpro impact pharmacophore modeling of N3 analogs?
Dynamic residues (e.g., E166, T190) alter pocket accessibility. To address this:
- Generate pharmacophores from MD ensemble-averaged structures.
- Include flexibility filters (e.g., RMSD < 2 Å) during molecular docking.
- Use FMO-PIEDA analysis to quantify residue-specific interaction energies across 1,000+ MD snapshots .
Q. What strategies reconcile discrepancies between computational binding scores and crystallographic data for N3 derivatives?
Discrepancies arise from force field limitations or solvation effects. Mitigation strategies include:
- Re-scoring docking poses with QM/MM-derived charges.
- Validating predictions via isothermal titration calorimetry (ITC) or surface plasmon resonance (SPR).
- Applying consensus scoring from multiple software (e.g., AutoDock, Glide) .
Q. Data Contradiction Analysis
- Frame-Dependent Binding Scores : While N3 shows stable binding in frames 1, 2, and 4 (ΔG < -10 kcal/mol), transient conformations (e.g., frame 9) exhibit weaker interactions. Researchers must prioritize frames with conserved hydrogen bonds (e.g., E166 salt bridge) to avoid overestimating affinity .
- False Positives in Virtual Screening : Compounds like almasilate score well computationally but lack practical utility due to poor bioavailability. Multi-parametric filtering (e.g., Lipinski’s rules, synthetic accessibility) is essential .
Q. Methodological Best Practices
- Crystallography : Use synchrotron radiation (λ = 0.97 Å) for high-resolution Mpro-N3 structures.
- SPE-MS Assays : Employ solid-phase extraction mass spectrometry to quantify covalent modification of Mpro by N3, distinguishing active-site binding from off-target reactions .
- PICO Framework : Structure research questions using Population (viral proteases), Intervention (N3 derivatives), Comparison (wild-type Mpro), and Outcome (ΔΔG, IC50) .
Properties
Molecular Formula |
C70H98N12O17 |
---|---|
Molecular Weight |
1379.6 g/mol |
IUPAC Name |
benzyl (E,4S)-4-[[(2S)-4-methyl-2-[[(2S)-3-methyl-2-[[(2S)-2-[(5-methyl-1,2-oxazole-3-carbonyl)amino]propanoyl]amino]butanoyl]amino]pentanoyl]amino]-5-[(3S)-2-oxopyrrolidin-3-yl]pent-2-enoate;hydrate |
InChI |
InChI=1S/2C35H48N6O8.H2O/c2*1-20(2)16-27(39-35(47)30(21(3)4)40-31(43)23(6)37-34(46)28-17-22(5)49-41-28)33(45)38-26(18-25-14-15-36-32(25)44)12-13-29(42)48-19-24-10-8-7-9-11-24;/h2*7-13,17,20-21,23,25-27,30H,14-16,18-19H2,1-6H3,(H,36,44)(H,37,46)(H,38,45)(H,39,47)(H,40,43);1H2/b2*13-12+;/t2*23-,25-,26+,27-,30-;/m00./s1 |
InChI Key |
FFGRVUOVZDZOBL-UHWYBZBWSA-N |
Isomeric SMILES |
CC1=CC(=NO1)C(=O)N[C@H](C(=O)N[C@H](C(=O)N[C@H](C(=O)N[C@H](/C=C/C(=O)OCC2=CC=CC=C2)C[C@H]3C(=O)NCC3)CC(C)C)C(C)C)C.CC1=CC(=NO1)C(=O)N[C@H](C(=O)N[C@H](C(=O)N[C@H](C(=O)N[C@H](/C=C/C(=O)OCC2=CC=CC=C2)C[C@H]3C(=O)NCC3)CC(C)C)C(C)C)C.O |
Canonical SMILES |
CC1=CC(=NO1)C(=O)NC(C)C(=O)NC(C(C)C)C(=O)NC(CC(C)C)C(=O)NC(CC2CCNC2=O)C=CC(=O)OCC3=CC=CC=C3.CC1=CC(=NO1)C(=O)NC(C)C(=O)NC(C(C)C)C(=O)NC(CC(C)C)C(=O)NC(CC2CCNC2=O)C=CC(=O)OCC3=CC=CC=C3.O |
Origin of Product |
United States |
Disclaimer and Information on In-Vitro Research Products
Please be aware that all articles and product information presented on BenchChem are intended solely for informational purposes. The products available for purchase on BenchChem are specifically designed for in-vitro studies, which are conducted outside of living organisms. In-vitro studies, derived from the Latin term "in glass," involve experiments performed in controlled laboratory settings using cells or tissues. It is important to note that these products are not categorized as medicines or drugs, and they have not received approval from the FDA for the prevention, treatment, or cure of any medical condition, ailment, or disease. We must emphasize that any form of bodily introduction of these products into humans or animals is strictly prohibited by law. It is essential to adhere to these guidelines to ensure compliance with legal and ethical standards in research and experimentation.