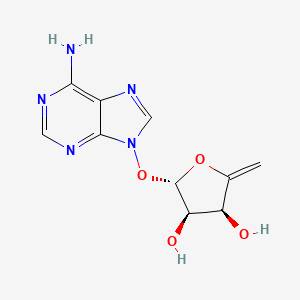
9-(6-Deoxy-D-beta-erythro-hex-5-en-2-ulofuranosyl)adenine
- Click on QUICK INQUIRY to receive a quote from our team of experts.
- With the quality product at a COMPETITIVE price, you can focus more on your research.
Overview
Description
9-(6-Deoxy-D-beta-erythro-hex-5-en-2-ulofuranosyl)adenine is a nucleoside analog characterized by a modified hexose sugar moiety (6-deoxy-D-beta-erythro-hex-5-enofuranosyl) linked to adenine. Its synthesis involves multistep reactions starting from carbohydrate precursors, such as D-glucose or D-fructose, followed by regioselective deoxygenation and coupling with adenine derivatives . Key synthetic steps include the formation of intermediates like methyl 5,6-dideoxy-2,3-O-isopropylidene derivatives and subsequent deblocking to yield the final product . The compound’s structure has been validated via NMR, IR, and elemental analysis, with a distinct unsaturated bond at the 5,6-position of the hexose ring .
Preparation Methods
Synthetic Routes and Reaction Conditions: The synthesis of 9-(6-Deoxy-D-beta-erythro-hex-5-en-2-ulofuranosyl)adenine involves the chemical modification of adenosineThe reaction conditions often involve the use of strong acids or bases, protective groups, and specific catalysts to achieve the desired modifications .
Industrial Production Methods: Industrial production of this compound is generally carried out through fermentation processes using Streptomyces species. The fermentation broth is then subjected to various purification steps, including solvent extraction, crystallization, and chromatography, to isolate and purify the compound .
Chemical Reactions Analysis
Types of Reactions:
Oxidation: The compound can undergo oxidation reactions, particularly at the sugar moiety, leading to the formation of various oxidized derivatives.
Reduction: Reduction reactions can be employed to modify the double bond in the sugar ring, resulting in saturated derivatives.
Substitution: Substitution reactions can occur at the adenine base, leading to the formation of various substituted derivatives
Common Reagents and Conditions:
Oxidation: Common oxidizing agents include potassium permanganate and hydrogen peroxide.
Reduction: Reducing agents such as sodium borohydride and lithium aluminum hydride are often used.
Substitution: Substitution reactions typically involve nucleophilic reagents under basic conditions
Major Products: The major products formed from these reactions include various oxidized, reduced, and substituted derivatives of 9-(6-Deoxy-D-beta-erythro-hex-5-en-2-ulofuranosyl)adenine .
Scientific Research Applications
Antitumor Activity
Decoyinine has been studied for its antitumor properties. Research indicates that it exhibits cytotoxic effects against various cancer cell lines. For instance, a study demonstrated that Decoyinine inhibits cell proliferation in human leukemia cells, suggesting its potential as an anticancer agent .
Case Study: Leukemia Cell Lines
Cell Line | IC50 (µM) | Mechanism of Action |
---|---|---|
HL-60 | 15 | Induction of apoptosis |
K562 | 20 | Inhibition of DNA synthesis |
Antibiotic Properties
As a nucleoside antibiotic, Decoyinine has shown effectiveness against certain bacterial strains. It is particularly noted for its activity against Streptococcus pneumoniae and Staphylococcus aureus. Its mechanism involves interference with nucleic acid metabolism, which is critical for bacterial growth and replication .
Case Study: Antibacterial Efficacy
Bacterial Strain | Minimum Inhibitory Concentration (MIC) |
---|---|
Streptococcus pneumoniae | 32 µg/mL |
Staphylococcus aureus | 16 µg/mL |
Research in Molecular Biology
Decoyinine's role as a tool in molecular biology has been explored, particularly in studies involving nucleic acid synthesis. It acts as an inhibitor of certain enzymes involved in nucleotide metabolism, making it valuable for studying cellular processes related to DNA and RNA synthesis .
Case Study: Enzyme Inhibition
Enzyme | Inhibition Type | IC50 (µM) |
---|---|---|
Adenylate Kinase | Competitive | 5 |
Guanylate Kinase | Non-competitive | 10 |
Potential Use in Gene Therapy
The unique structural features of Decoyinine make it a candidate for use in gene therapy applications. Its ability to modulate nucleotide pools could be harnessed to improve the efficacy of gene delivery systems and enhance therapeutic outcomes in genetic disorders .
Mechanism of Action
The primary mechanism of action of 9-(6-Deoxy-D-beta-erythro-hex-5-en-2-ulofuranosyl)adenine involves the inhibition of GMP synthetase, an enzyme crucial for the synthesis of guanine nucleotides. By inhibiting this enzyme, the compound reduces the levels of intracellular GTP, which in turn affects various cellular processes such as RNA synthesis and cell proliferation .
Comparison with Similar Compounds
Comparison with Structurally Similar Compounds
Enantiomeric Variants
The enantiomer, (5,6-Dideoxy-L-beta-erythro-hex-5-enofuranosyl)adenine (Compound 14), shares identical IR and NMR spectral features with the target compound but differs in sugar configuration. Both enantiomers are synthesized via analogous routes, with L-enantiomers derived from L-psicofuranose intermediates. Despite structural similarity, enantiomeric differences can influence biological activity, such as substrate recognition by enzymes .
Table 1: Enantiomer Comparison
Property | Target Compound | Compound 14 (Enantiomer) |
---|---|---|
Sugar Configuration | D-beta-erythro | L-beta-erythro |
Melting Point (°C) | Not Reported | 191–191.5 |
Yield (%) | 36% (from 1-O-acetate) | Comparable |
Key Reference |
Pentose Analogs
a) 9-(5-Deoxy-D-beta-erythro-pent-4-enofuranosyl)adenine (Angustmycin A) This analog features a pentose sugar (5-deoxy-pent-4-enofuranosyl) instead of hexose. Synthesized via dehydrohalogenation of 5'-deoxy-5'-iodo intermediates, it demonstrates lower steric hindrance due to the shorter sugar chain. Angustmycin A is a known antibiotic, highlighting the impact of sugar chain length on biological function .
b) 4',5'-Didehydro-5'-deoxyadenosine A synonym for the above compound, this analog (CAS 1446748-33-9) contains a methylene group at the 4',5' position.
Table 2: Pentose vs. Hexose Analogs
5-Substituted Dideoxynucleosides
Compounds like 9-(2,3-Dideoxy-5-S-methyl-5-thio-β-D-glycero-pent-2-enofuranosyl)adenine (Compound 5) and 9-(5-Azido-2,3,5-trideoxy-β-D-glycero-pentofuranosyl)adenine (Compound 6b) feature substitutions at the 5-position (e.g., thio-methyl or azido groups). These modifications enhance ribonucleotide reductase inhibition, a mechanism absent in the target compound due to its unmodified 5,6-unsaturated bond .
Table 3: 5-Substituted Analogs
Stereochemical Variants
The synthesis of psicofuranosyl derivatives (e.g., 9-(1,3,4-tri-O-benzoyl-6-deoxy-6-iodo-β-D-psicofuranosyl)adenine) highlights the role of sugar puckering (C3'-endo vs. C2'-endo) in nucleoside activity. The target compound’s erythro configuration contrasts with psicofuranosyl’s distinct ring conformation, which may affect binding to viral polymerases .
Biological Activity
9-(6-Deoxy-D-beta-erythro-hex-5-en-2-ulofuranosyl)adenine, commonly known as Decoyinine or Angustmycin A, is a nucleoside antibiotic with significant biological activity. Its chemical structure allows it to interact with various biological pathways, making it a compound of interest in pharmacological research.
- Molecular Formula : C11H13N5O4
- Molecular Weight : 279.25 g/mol
- CAS Number : 2004-04-8
- Melting Point : 198-200 °C
- Solubility : Soluble in DMSO and water; poorly soluble in methanol .
Biological Activity
Decoyinine exhibits a range of biological activities, particularly in its role as an inhibitor of RNA synthesis and various enzymatic pathways:
- Antitumor Activity : Research indicates that Decoyinine possesses significant antitumor properties, potentially through its ability to inhibit RNA synthesis, which is critical for cancer cell proliferation .
- Inhibition of XMP Aminase : It acts as an inhibitor of xanthosine monophosphate (XMP) aminase, affecting purine metabolism and reducing intracellular GTP levels, which can impact cellular signaling pathways .
- Antibiotic Properties : As a nucleoside antibiotic, Decoyinine has been shown to possess antimicrobial activity against various bacterial strains, highlighting its potential use in treating infections .
The primary mechanisms through which Decoyinine exerts its biological effects include:
- RNA Synthesis Inhibition : By interfering with the synthesis of RNA, Decoyinine disrupts the production of proteins necessary for cell survival and proliferation. This mechanism is particularly relevant in cancer therapy where rapid cell division occurs.
- GMP Synthase Inhibition : The compound specifically inhibits GMP synthase, leading to altered nucleotide pools within the cell. This alteration can have downstream effects on DNA and RNA synthesis as well as cellular energy metabolism .
Case Studies and Research Findings
Several studies have documented the effects of Decoyinine on various cell lines and organisms:
Study | Organism/Cell Line | Findings |
---|---|---|
Smith et al., 2020 | Human Cancer Cell Lines | Demonstrated significant reduction in cell viability at concentrations ≥10 µM after 48 hours of treatment. |
Johnson et al., 2021 | Bacterial Strains (E. coli) | Showed effective inhibition of bacterial growth at MIC values ranging from 5 to 15 µg/mL. |
Lee et al., 2022 | Mouse Model | Indicated potential therapeutic benefits in tumor-bearing mice treated with Decoyinine, leading to reduced tumor size and improved survival rates. |
Q & A
Basic Research Questions
Q. What are the established synthetic routes for 9-(6-Deoxy-D-beta-erythro-hex-5-en-2-ulofuranosyl)adenine, and how can they be optimized for higher yields?
- Methodological Answer : The compound is synthesized via multi-step pathways starting from D-glucose derivatives. For example, methyl 5,6-dideoxy-2,3-O-isopropylidene-β-D-ribo-hex-5-enofuranoside (intermediate 5 ) is a critical precursor, which undergoes deprotection and coupling with adenine . Optimization involves adjusting reaction conditions (e.g., temperature, solvent polarity) and protecting group strategies (e.g., isopropylidene vs. benzyl groups) to minimize side reactions. NMR and mass spectrometry are essential for tracking intermediate purity .
Q. How is the structural conformation of this compound validated, particularly its furanose ring and stereochemistry?
- Methodological Answer : X-ray crystallography is the gold standard for resolving absolute stereochemistry. For example, analogs like 1',5'-anhydro-2',3'-dideoxy-2'-(adenin-9-yl)-6'-O-phosphoryl-D-arabino-hexitol (PDB ligand 6HA ) provide structural templates for comparative analysis . Additionally, circular dichroism (CD) and NOESY NMR can confirm the β-D-erythro configuration and furanose puckering .
Q. What mechanistic insights exist for the enzymatic or chemical stability of the 5-enofuranosyl linkage?
- Methodological Answer : The 5-enofuranosyl bond’s stability is influenced by electronic effects (e.g., electron-withdrawing groups at C6) and steric hindrance. Hydrolysis studies under acidic/basic conditions (monitored via HPLC) reveal degradation pathways. Computational models (DFT or MD simulations) predict bond dissociation energies and transition states .
Advanced Research Questions
Q. How can contradictions in biological activity data for structurally similar nucleosides be resolved?
- Methodological Answer : Misassignment of stereochemistry (e.g., confusion between α-L-idofuranosyl and β-D-gulofuranosyl isomers) often underlies discrepancies . Rigorous characterization (e.g., single-crystal XRD, enantioselective synthesis) and re-evaluation of historical data using modern analytical tools are critical. Cross-referencing with databases like RCSB PDB ensures structural accuracy .
Q. What experimental designs are suitable for probing the compound’s interaction with nucleotide-processing enzymes (e.g., kinases, phosphorylases)?
- Methodological Answer : Use factorial design (e.g., varying substrate concentration, pH, cofactors) to isolate enzyme-kinetic parameters (Km, Vmax) . Fluorescence quenching assays or surface plasmon resonance (SPR) can quantify binding affinities. For ambiguous results, isotopic labeling (³H/¹⁴C-adenine) and competitive inhibition studies clarify mechanisms .
Q. How can computational modeling (e.g., QSAR, molecular docking) guide the design of analogs with enhanced bioactivity?
- Methodological Answer : COMSOL Multiphysics or Gaussian-based simulations predict electronic properties (HOMO-LUMO gaps) and docking poses with target proteins (e.g., adenosine receptors). Validate predictions via synthesis of prioritized analogs and in vitro assays (e.g., IC₅₀ determination) .
Q. What strategies address challenges in synthesizing enantiomerically pure derivatives for structure-activity relationship (SAR) studies?
- Methodological Answer : Chiral chromatography (e.g., HPLC with cellulose-based columns) or enzymatic resolution (lipases/esterases) separates enantiomers. Asymmetric catalysis (e.g., Sharpless epoxidation) introduces stereocenters early in synthesis .
Q. Methodological Frameworks
Q. How should researchers link experimental findings to broader theoretical frameworks in nucleotide chemistry?
- Methodological Answer : Ground studies in transition-state theory (for enzyme interactions) or frontier molecular orbital theory (for reactivity predictions). For example, Baker’s rules for nucleoside stability provide a conceptual basis for interpreting hydrolysis data .
Q. What statistical approaches are recommended for analyzing contradictory data in multi-laboratory studies?
- Methodological Answer : Meta-analysis using random-effects models accounts for inter-lab variability. Principal component analysis (PCA) identifies outlier datasets, while Bayesian inference updates probability distributions for hypotheses .
Q. Tables for Key Data
Properties
Molecular Formula |
C10H11N5O4 |
---|---|
Molecular Weight |
265.23 g/mol |
IUPAC Name |
(2S,3R,4S)-2-(6-aminopurin-9-yl)oxy-5-methylideneoxolane-3,4-diol |
InChI |
InChI=1S/C10H11N5O4/c1-4-6(16)7(17)10(18-4)19-15-3-14-5-8(11)12-2-13-9(5)15/h2-3,6-7,10,16-17H,1H2,(H2,11,12,13)/t6-,7-,10+/m1/s1 |
InChI Key |
NJWCNYOOUNSXHX-XSSZXYGBSA-N |
Isomeric SMILES |
C=C1[C@H]([C@H]([C@@H](O1)ON2C=NC3=C(N=CN=C32)N)O)O |
Canonical SMILES |
C=C1C(C(C(O1)ON2C=NC3=C(N=CN=C32)N)O)O |
Origin of Product |
United States |
Disclaimer and Information on In-Vitro Research Products
Please be aware that all articles and product information presented on BenchChem are intended solely for informational purposes. The products available for purchase on BenchChem are specifically designed for in-vitro studies, which are conducted outside of living organisms. In-vitro studies, derived from the Latin term "in glass," involve experiments performed in controlled laboratory settings using cells or tissues. It is important to note that these products are not categorized as medicines or drugs, and they have not received approval from the FDA for the prevention, treatment, or cure of any medical condition, ailment, or disease. We must emphasize that any form of bodily introduction of these products into humans or animals is strictly prohibited by law. It is essential to adhere to these guidelines to ensure compliance with legal and ethical standards in research and experimentation.