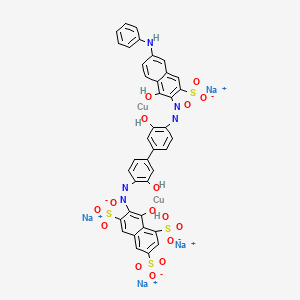
Cuprate
- Click on QUICK INQUIRY to receive a quote from our team of experts.
- With the quality product at a COMPETITIVE price, you can focus more on your research.
Overview
Description
Cuprates are copper-oxide compounds that revolutionized condensed matter physics with their high-temperature superconductivity (HTSC), first discovered in 1986 . Their parent compounds, such as La₂CuO₄, are antiferromagnetic (AF) Mott insulators with quasi-two-dimensional (2D) layered structures composed of CuO₂ planes . Doping these materials with charge carriers (holes or electrons) suppresses AF order and induces superconductivity, with critical temperatures (Tc) up to 133 K . Key features include:
- d-wave pairing symmetry: The superconducting gap has nodes, distinct from conventional s-wave superconductors .
- Pseudogap phenomenon: A partial energy gap persists above Tc in the underdoped regime .
- Strong electronic correlations: Charge-transfer insulators with effective Hubbard interaction U ~3 eV .
Preparation Methods
Synthetic Routes and Reaction Conditions: Cuprates can be synthesized through various methods, including solid-state reactions and sol-gel processes. One common method involves the reaction of copper salts with alkali metal oxides or hydroxides under high-temperature conditions. For example, yttrium barium copper oxide (YBa₂Cu₃O₇) can be prepared by mixing yttrium oxide, barium carbonate, and copper oxide, followed by heating the mixture at high temperatures in an oxygen atmosphere .
Industrial Production Methods: Industrial production of cuprates often involves large-scale solid-state reactions. The raw materials, such as copper oxide and other metal oxides, are mixed and heated in large furnaces. The process requires precise control of temperature and oxygen levels to ensure the formation of the desired cuprate phase .
Chemical Reactions Analysis
Types of Reactions: Cuprates undergo various chemical reactions, including oxidation, reduction, and substitution reactions. They are known to participate in nucleophilic addition reactions, particularly with organocuprates (Gilman reagents), which are useful in organic synthesis .
Common Reagents and Conditions: Common reagents used in reactions with cuprates include alkyllithium compounds, which react with copper salts to form organocuprates. These reactions typically occur under anhydrous conditions to prevent hydrolysis of the reagents .
Major Products Formed: The major products formed from reactions involving cuprates depend on the specific reagents and conditions used. For example, the reaction of an organocuprate with an alkyl halide can yield a new carbon-carbon bond, forming a substituted alkane .
Scientific Research Applications
Cuprates have a wide range of scientific research applications, particularly in the field of superconductivity. High-temperature cuprate superconductors are used in various applications, including magnetic resonance imaging (MRI) machines, particle accelerators, and power transmission lines . Additionally, cuprates are studied for their potential use in electronic devices, such as transistors and sensors .
Mechanism of Action
The mechanism by which cuprates exhibit superconductivity is not fully understood. it is believed that the superconducting properties arise from the interaction of electrons within the copper oxide planes. The exact nature of this interaction is still a topic of ongoing research, with theories suggesting the involvement of spin fluctuations or phonons .
Comparison with Similar Compounds
Iron-Based Superconductors (FeAs/FeSe)
Iron-based superconductors (pnictides) share layered structures and phase diagrams with cuprates but exhibit distinct microscopic mechanisms:
Key Similarities :
- Layered structures with active FeAs/FeSe or CuO₂ planes .
- Phase diagrams with adjacent AF and superconducting phases .
Key Differences :
- Fe-based compounds involve orbital-selective correlations and multiband physics, unlike cuprates .
- Pressure and doping effects are more pronounced in Fe-based systems .
Strontium Iridate (Sr₂IrO₄)
Sr₂IrO₄ is isostructural to La₂CuO₄ but lacks superconductivity despite electronic similarities:
- Electronic Structure : Quasi-2D with spin-orbit-coupled Jeff = 1/2 states, mimicking cuprate Fermi arcs .
- Magnetic Order : AF below TN = 240 K .
- Doping Response : Surface electron doping induces pseudogap and Fermi arcs, but bulk superconductivity remains elusive .
Table : Sr₂IrO₄ vs. La₂CuO₄
Nickelates (RNiO₂)
Nickelates (e.g., NdNiO₂) are the closest structural analogs to cuprates but differ in electronic structure:
- Parent State : Correlated metal with larger Hubbard U (~6 eV) .
- Doping Response: Limited Tc (~15 K) compared to cuprates .
- Key Distinction : Ni³⁺ in nickelates vs. Cu²⁺ in cuprates; weaker AF exchange in nickelates .
AFeSe₂ (A = Tl, K, Rb, Cs)
AFeSe₂ compounds mimic this compound parent compounds with FeSe₂ layers analogous to CuO₂ planes:
- Structure: FeSe₂ layers are isoelectronic to CuO₂ planes but with non-coplanar Se atoms .
- Magnetic Order : Néel AF with exchange interactions comparable to cuprates .
- Potential for SC: Theoretical predictions suggest high Tc upon doping, though experimental confirmation is pending .
Electron-Doped vs. Hole-Doped Cuprates
Electron-doped cuprates (e.g., Nd₂₋ₓCeₓCuO₄) exhibit distinct phase diagrams:
- Charge Ordering : Observed at higher temperatures than in hole-doped systems .
- Pseudogap: Less pronounced, with coexisting AF fluctuations .
Data Tables
Table 1: Parent Compound Properties
Table 2: Superconducting Properties
Biological Activity
Cuprates are a class of compounds that contain copper and exhibit a wide range of biological activities. These compounds have garnered significant interest due to their potential applications in medicine, particularly in the fields of oncology and neurobiology. This article focuses on the biological activity of cuprates, highlighting their mechanisms of action, therapeutic potentials, and relevant case studies.
Cuprates exhibit various biological activities through several mechanisms:
- Antimicrobial Activity : Cuprates have demonstrated effectiveness against a range of pathogens, including bacteria and fungi. Their antimicrobial properties are often attributed to the release of reactive oxygen species (ROS) upon interaction with biological membranes.
- Antitumor Effects : Certain cuprate complexes have shown promise in inhibiting tumor growth. For instance, copper(II) complexes have been observed to induce apoptosis in cancer cells by disrupting mitochondrial function and promoting oxidative stress.
- Neuroprotective Properties : Some studies suggest that this compound compounds can protect neuronal cells from oxidative damage, potentially offering therapeutic benefits in neurodegenerative diseases.
Case Studies
-
Copper(II) N-salicylideneaminoacidato Complexes :
- A study investigated the effects of these complexes on freshwater algae, specifically Chlorella vulgaris. It was found that these cuprates significantly reduced chlorophyll content and inhibited photosynthetic electron transport, indicating potential ecological impacts as well as therapeutic applications in controlling algal blooms .
- Titanocene Dichlorides :
- Perylenequinones Related to Cuprates :
Summary of Biological Activities
Activity Type | Description | Examples |
---|---|---|
Antimicrobial | Effective against bacteria and fungi | Copper(II) complexes |
Antitumor | Induces apoptosis in cancer cells | Titanocene dichlorides |
Neuroprotective | Protects neurons from oxidative stress | Copper(II) N-salicylideneaminoacidato complexes |
Synthesis and Evaluation
The synthesis of this compound compounds often involves complex organic reactions such as conjugate additions. For example, this compound-mediated reactions have been utilized to create biologically active cyclopropyl- and tert-butylfarnesyl diphosphate analogs, which were evaluated for their activity against specific cellular targets .
Toxicological Considerations
While cuprates exhibit promising biological activities, it is essential to consider their toxicity profiles. Research indicates that certain this compound compounds can lead to cellular damage at high concentrations, necessitating careful evaluation during therapeutic development .
Q & A
Basic Research Questions
Q. What experimental methodologies are essential for synthesizing high-quality cuprate superconductors?
- Methodological Answer : this compound synthesis requires precise control of stoichiometry, oxygen doping, and annealing conditions. Solid-state reactions involve mixing precursor oxides (e.g., CuO, BaO) and calcining at 900–1000°C in controlled atmospheres. Post-annealing in oxygen flow optimizes carrier density . Characterization techniques like X-ray diffraction (XRD) and transmission electron microscopy (TEM) validate phase purity and crystal structure .
Q. How do researchers determine the critical temperature (Tc) of this compound superconductors?
- Methodological Answer : Tc is measured via resistivity, magnetic susceptibility (DC/AC), and specific heat experiments. Resistivity drops to zero at Tc, while diamagnetic shielding in susceptibility confirms bulk superconductivity. Consistent cooling rates and sample encapsulation prevent oxygen loss during measurements .
Q. What are the key structural features of cuprates that influence superconducting properties?
- Methodological Answer : Layered perovskite structures with CuO2 planes are critical. Hole or electron doping via chemical substitution (e.g., Sr in La2−xSrxCuO4) modulates carrier concentration. Neutron diffraction and EXAFS (Extended X-ray Absorption Fine Structure) analyze lattice distortions and dopant distribution .
Advanced Research Questions
Q. How can angle-resolved photoemission spectroscopy (ARPES) resolve controversies about the this compound Fermi surface and pseudogap?
- Methodological Answer : ARPES with ≤2 meV energy and 0.2° angular resolution maps electronic bands near the Fermi level. Data interpretation requires distinguishing between pseudogap (static charge/orbital order) and superconducting gap (d-wave symmetry). Comparative studies across doping levels (underdoped to overdoped) isolate pseudogap contributions .
Q. What strategies address contradictory data on electronic inhomogeneity in cuprates?
- Methodological Answer : Scanning tunneling microscopy (STM) and μ-ARPES (micron-scale ARPES) identify nanoscale phase separation. Statistical analysis of local density of states (LDOS) variations and machine learning clustering algorithms quantify spatial inhomogeneity. Cross-validation with bulk-sensitive techniques (e.g., NMR) reconciles local vs. averaged properties .
Q. How do researchers differentiate between competing theories (e.g., spin-fluctuation vs. phonon-mediated pairing) for this compound superconductivity?
- Methodological Answer : Neutron scattering detects spin resonance modes near (π, π), supporting spin-fluctuation models. Isotope effect studies (replacing <sup>16</sup>O with <sup>18</sup>O) test phonon contributions. Multidisciplinary approaches combining transport, spectroscopy, and theoretical modeling (e.g., DFT+DMFT) are critical .
Q. What systematic frameworks ensure reproducibility in this compound thin-film experiments?
- Methodological Answer : Standardize substrate preparation (e.g., SrTiO3 annealing), deposition parameters (laser fluence, oxygen pressure), and post-growth annealing. Publish raw data (e.g., XRD spectra, RHEED patterns) and metadata (growth logs) in open repositories. Collaborative inter-lab studies validate protocols .
Q. Data Analysis and Interpretation
Q. How should researchers handle conflicting results about the pseudogap’s role in superconductivity?
- Methodological Answer : Conduct meta-analyses of ARPES, STM, and quantum oscillation datasets. Apply Bayesian statistics to weigh evidence for pseudogap as a precursor vs. competing order. Transparent reporting of experimental conditions (e.g., doping, temperature) reduces bias .
Q. What computational tools are used to model this compound electronic structure and phase diagrams?
- Methodological Answer : Density Functional Theory (DFT) with Hubbard U corrections (DFT+U) models correlated electrons. Dynamical Mean-Field Theory (DMFT) integrates local quantum fluctuations. Open-source codes (e.g., Quantum ESPRESSO) enable reproducibility. Validation against ARPES and neutron data ensures accuracy .
Q. Tables of Key Findings
Q. Guidelines for Rigorous Research
- Data Integrity : Archive raw datasets (e.g., spectroscopy curves, diffraction patterns) with timestamps and calibration details .
- Collaboration : Involve specialists (e.g., statisticians for meta-analysis, methodologists for bias reduction) .
- Transparency : Pre-register hypotheses and analysis plans to avoid post hoc rationalization .
Properties
CAS No. |
72927-72-1 |
---|---|
Molecular Formula |
C38H23Cu2N5Na4O16S4 |
Molecular Weight |
1152.9 g/mol |
IUPAC Name |
tetrasodium;7-[[4-[4-[(6-anilino-1-hydroxy-3-sulfonatonaphthalen-2-yl)diazenyl]-3-hydroxyphenyl]-2-hydroxyphenyl]diazenyl]-8-hydroxynaphthalene-1,3,6-trisulfonate;copper |
InChI |
InChI=1S/C38H27N5O16S4.2Cu.4Na/c44-29-14-19(6-10-27(29)40-42-35-32(62(54,55)56)16-21-12-24(8-9-26(21)37(35)46)39-23-4-2-1-3-5-23)20-7-11-28(30(45)15-20)41-43-36-33(63(57,58)59)17-22-13-25(60(48,49)50)18-31(61(51,52)53)34(22)38(36)47;;;;;;/h1-18,39,44-47H,(H,48,49,50)(H,51,52,53)(H,54,55,56)(H,57,58,59);;;;;;/q;;;4*+1/p-4 |
InChI Key |
FGMABLXDGUWFNG-UHFFFAOYSA-J |
Canonical SMILES |
C1=CC=C(C=C1)NC2=CC3=CC(=C(C(=C3C=C2)O)N=NC4=C(C=C(C=C4)C5=CC(=C(C=C5)N=NC6=C(C7=C(C=C(C=C7C=C6S(=O)(=O)[O-])S(=O)(=O)[O-])S(=O)(=O)[O-])O)O)O)S(=O)(=O)[O-].[Na+].[Na+].[Na+].[Na+].[Cu].[Cu] |
Origin of Product |
United States |
Disclaimer and Information on In-Vitro Research Products
Please be aware that all articles and product information presented on BenchChem are intended solely for informational purposes. The products available for purchase on BenchChem are specifically designed for in-vitro studies, which are conducted outside of living organisms. In-vitro studies, derived from the Latin term "in glass," involve experiments performed in controlled laboratory settings using cells or tissues. It is important to note that these products are not categorized as medicines or drugs, and they have not received approval from the FDA for the prevention, treatment, or cure of any medical condition, ailment, or disease. We must emphasize that any form of bodily introduction of these products into humans or animals is strictly prohibited by law. It is essential to adhere to these guidelines to ensure compliance with legal and ethical standards in research and experimentation.