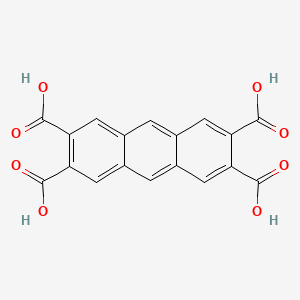
Anthracene-2,3,6,7-tetracarboxylic acid
- Click on QUICK INQUIRY to receive a quote from our team of experts.
- With the quality product at a COMPETITIVE price, you can focus more on your research.
Overview
Description
Anthracene-2,3,6,7-tetracarboxylic acid is a highly functionalized anthracene derivative with four carboxylic acid groups at the 2, 3, 6, and 7 positions of the aromatic core. This compound has garnered attention for its unique structural rigidity, electronic properties, and versatility in polymer chemistry and materials science.
Synthesis:
Historically, the synthesis of 2,3,6,7-substituted anthracene derivatives was challenging due to the low reactivity of these positions compared to the 1,4,5,8 and 9,10 sites. Early methods required multiple steps and costly reagents, resulting in low yields . However, recent advancements have streamlined the synthesis into three steps using cost-effective starting materials (e.g., o-xylene and phthalic anhydride), achieving high yields (>90%) and purity (>98%) via oxidation with potassium permanganate and dehydration with acetic anhydride .
Applications:
The compound serves as a precursor for dianhydrides (e.g., 2,3,6,7-anthracenetetracarboxylic dianhydride), which are critical for synthesizing polyimides with exceptional thermal stability (Tg > 400°C) and fluorescence properties . Its incorporation into polymers enables Diels-Alder crosslinking reactions, making it valuable for creating heat-resistant, mechanically robust networks .
Preparation Methods
Synthetic Routes and Reaction Conditions: Anthracene-2,3,6,7-tetracarboxylic acid can be synthesized through several methods. One common approach involves the oxidation of anthracene derivatives. For instance, the oxidation of anthracene-2,3,6,7-tetramethyl with potassium permanganate in an alkaline medium yields this compound . Another method involves the cycloaddition reactions of anthracene derivatives with maleic anhydride, followed by hydrolysis to obtain the tetracarboxylic acid .
Industrial Production Methods: Industrial production of this compound typically involves large-scale oxidation processes. These processes often use strong oxidizing agents such as potassium permanganate or nitric acid under controlled conditions to ensure high yields and purity .
Chemical Reactions Analysis
Types of Reactions: Anthracene-2,3,6,7-tetracarboxylic acid undergoes various chemical reactions, including:
Oxidation: The compound can be further oxidized to form quinones and other oxidized derivatives.
Reduction: Reduction reactions can convert the carboxyl groups to hydroxyl groups or other reduced forms.
Substitution: The aromatic ring of this compound can undergo electrophilic substitution reactions, such as nitration and halogenation.
Common Reagents and Conditions:
Oxidation: Potassium permanganate, nitric acid, and other strong oxidizing agents are commonly used.
Reduction: Reducing agents like lithium aluminum hydride (LiAlH₄) or sodium borohydride (NaBH₄) are employed.
Substitution: Electrophilic reagents such as nitric acid for nitration and halogens for halogenation are used.
Major Products Formed:
Oxidation: Quinones and other oxidized derivatives.
Reduction: Hydroxylated derivatives.
Substitution: Nitrated or halogenated anthracene derivatives.
Scientific Research Applications
Chemical Applications
Synthesis of Organic Compounds
ATCA serves as a precursor for synthesizing various organic compounds, including dyes and polymers. Its structure allows it to undergo multiple chemical reactions such as oxidation, reduction, and substitution. For instance:
- Oxidation : ATCA can be oxidized to form quinones and other derivatives using agents like potassium permanganate.
- Reduction : The carboxyl groups can be reduced to hydroxyl groups using reducing agents like lithium aluminum hydride.
- Substitution : The aromatic ring can participate in electrophilic substitution reactions, producing nitrated or halogenated derivatives.
These reactions make ATCA valuable in developing new materials and compounds in organic chemistry.
Biological Applications
Photodynamic Therapy
ATCA has shown promise in photodynamic therapy (PDT), where it generates reactive oxygen species (ROS) upon light activation. This property enables it to selectively target and destroy cancer cells while minimizing damage to surrounding healthy tissue. A study indicated that ATCA effectively induces apoptosis in cancer cells when activated by specific wavelengths of light, outperforming other anthracene derivatives due to its optimal carboxylic group arrangement.
Antioxidant Properties
Research has demonstrated that ATCA exhibits significant antioxidant activity, helping neutralize free radicals and protect cells from oxidative stress. In vitro assays showed that ATCA could scavenge free radicals more efficiently than some traditional antioxidants by donating hydrogen atoms from its carboxyl groups.
Antimicrobial Activity
Preliminary studies suggest that ATCA possesses antimicrobial properties against various pathogens. Tests against Staphylococcus aureus and Escherichia coli revealed significant inhibitory effects, with minimum inhibitory concentrations comparable to established antibiotics. This suggests potential applications for developing new antimicrobial agents based on ATCA's structure.
Industrial Applications
ATCA is utilized in producing high-performance materials such as polyimides and other advanced polymers. Its unique structure allows for the formation of stable complexes with metal ions, enhancing its reactivity and solubility in various industrial processes.
Comparative Analysis of Biological Activities
The following table summarizes the biological activities of ATCA compared to other anthracene derivatives:
Compound Name | Photodynamic Activity | Antioxidant Activity | Antimicrobial Activity |
---|---|---|---|
Anthracene-2,3,6,7-tetracarboxylic acid | High | Moderate | Significant |
9,10-Anthraquinone | Moderate | Low | High |
2-Hydroxyanthraquinone | Low | High | Moderate |
This comparison highlights ATCA's unique position among anthracene derivatives due to its superior photodynamic activity and significant antimicrobial potential.
Case Study 1: Photodynamic Applications
A study demonstrated that ATCA could effectively induce apoptosis in cancer cells when activated by specific wavelengths of light. The generation of ROS led to cellular damage and death, showcasing its potential as a therapeutic agent in cancer treatment.
Case Study 2: Antioxidant Mechanism
In vitro assays indicated that ATCA could scavenge free radicals more efficiently than some traditional antioxidants. The mechanism involved the donation of hydrogen atoms from the carboxyl groups, which neutralized reactive species and reduced cellular damage.
Case Study 3: Antimicrobial Studies
In tests against Staphylococcus aureus and Escherichia coli, ATCA demonstrated significant inhibitory effects with minimum inhibitory concentrations comparable to established antibiotics. These findings suggest potential applications in developing new antimicrobial agents based on ATCA's structure.
Mechanism of Action
The mechanism of action of anthracene-2,3,6,7-tetracarboxylic acid involves its ability to interact with various molecular targets and pathways. The compound’s carboxyl groups can form hydrogen bonds and electrostatic interactions with proteins and other biomolecules, influencing their structure and function . Additionally, its aromatic ring system allows for π-π stacking interactions, which can affect the compound’s binding affinity and specificity .
Comparison with Similar Compounds
To contextualize its utility, anthracene-2,3,6,7-tetracarboxylic acid is compared to structurally or functionally analogous compounds, including perylene tetracarboxylic dianhydride (PTCDA), pyromellitic dianhydride (PMDA), and fluorinated dianhydrides (e.g., 8FDA).
Table 1: Key Properties of this compound and Analogues
Structural and Functional Insights :
Reactivity and Substitution Patterns :
- This compound’s substitution pattern enhances steric rigidity, promoting thermal stability comparable to fluorinated dianhydrides like 8FDA . In contrast, PTCDA’s planar perylene core facilitates π-π stacking, ideal for charge transport in organic electronics but limits solubility .
Thermal and Mechanical Performance: Polyimides derived from this compound exhibit Tg values (~401°C) rivaling 8FDA-based polymers, but with superior solubility due to non-fluorinated ester intermediates . PMDA-based polyimides, while thermally robust, suffer from brittleness and poor processability .
Synthetic Accessibility :
- This compound’s synthesis is more efficient than PTCDA’s, which involves complex cyclization steps . However, fluorinated analogues like 8FDA require hazardous reagents (e.g., DAST), complicating scale-up .
Table 2: Comparative Advantages and Limitations
Biological Activity
Anthracene-2,3,6,7-tetracarboxylic acid (ATCA) is a polycarboxylic aromatic compound characterized by its four carboxylic acid groups attached to an anthracene backbone. Its molecular formula is C14H10O8, and it has gained attention for its diverse biological activities and potential applications in fields such as photodynamic therapy (PDT), drug delivery, and material science.
ATCA's structure enables it to form stable coordination complexes with various metal ions, enhancing its reactivity and solubility in biological systems. The presence of multiple carboxylic groups contributes to its ability to interact with biological molecules and metal ions, making it a versatile compound for research.
Biological Activities
Research indicates that ATCA exhibits several significant biological activities:
- Photodynamic Therapy : ATCA can generate reactive oxygen species (ROS) upon light activation, which is crucial for its application in cancer treatment. This property allows it to selectively target and destroy cancer cells while minimizing damage to surrounding healthy tissue.
- Antioxidant Properties : Studies have shown that ATCA exhibits antioxidant activity, helping to neutralize free radicals and protect cells from oxidative stress.
- Antimicrobial Activity : Preliminary studies suggest that ATCA may possess antimicrobial properties against various pathogens. Its structural similarity to other anthraquinone derivatives, known for their antibacterial effects, supports this potential .
Case Studies
- Photodynamic Applications : A study demonstrated that ATCA could effectively induce apoptosis in cancer cells when activated by specific wavelengths of light. This was attributed to the generation of ROS that led to cellular damage and death. The effectiveness of ATCA in PDT was compared with other anthracene derivatives, highlighting its superior performance due to the optimal arrangement of carboxylic groups.
- Antioxidant Mechanism : In vitro assays indicated that ATCA could scavenge free radicals more efficiently than some traditional antioxidants. The mechanism involved the donation of hydrogen atoms from the carboxyl groups, which neutralized reactive species and reduced cellular damage .
- Antimicrobial Studies : In tests against Staphylococcus aureus and Escherichia coli, ATCA demonstrated significant inhibitory effects, with minimum inhibitory concentrations (MICs) comparable to established antibiotics. These findings suggest potential applications in developing new antimicrobial agents based on ATCA's structure .
Comparative Analysis
The following table summarizes the biological activities of ATCA compared to other anthracene derivatives:
Compound Name | Photodynamic Activity | Antioxidant Activity | Antimicrobial Activity |
---|---|---|---|
This compound | High | Moderate | Significant |
9,10-Anthraquinone | Moderate | Low | High |
2-Hydroxyanthraquinone | Low | High | Moderate |
Q & A
Basic Research Questions
Q. What are the optimized synthetic routes for Anthracene-2,3,6,7-tetracarboxylic acid, and how do they address historical challenges?
The compound is synthesized in three steps via a modified protocol that improves upon older methods requiring costly reagents and low yields. Key innovations include:
- Use of ester/acid chloride intermediates to enhance solubility and polymerizability of precursors .
- Hydrolysis of anthracene diimide in NaOH to yield the tetracarboxylic acid, followed by purification via potassium salt formation .
- Avoidance of anthraquinone formation during oxidation steps, a common issue in earlier syntheses . Challenges like precursor insolubility were mitigated by optimizing reaction conditions (e.g., solvent selection and temperature).
Q. How can solubility issues of this compound derivatives be addressed during polymerization?
Solubility is improved by:
- Synthesizing ester derivatives (e.g., tetrakis(methoxycarbonyl)anthracene) for better solubility in organic solvents .
- Converting the acid to its dianhydride form using triethylamine and phosgene in methylene chloride, enabling solution-phase chemistry for polyimide synthesis .
- Employing potassium salts of the acid for aqueous-phase reactions, though direct characterization remains challenging due to limited solubility .
Q. What characterization techniques are most effective for verifying the purity and structure of this compound?
Key methods include:
- NMR spectroscopy : 1H and 13C NMR in DMSO-d₆ confirm esterification and anhydride formation (e.g., δ 3.91 ppm for methoxy groups in tetramethyl esters) .
- Mass spectrometry : FAB-MS provides molecular ion peaks (e.g., m/z 410 for tetramethyl esters) .
- Thermal analysis : TGA reveals thermal stability of polymers derived from the acid, with decomposition temperatures exceeding 300°C .
Advanced Research Questions
Q. How can syn/anti isomer separation be achieved during monomer synthesis, and what impact does isomerism have on polymer properties?
Isomer separation remains unresolved in current methods. Potential strategies include:
- Screening ester derivatives (e.g., propyl vs. methyl esters) for crystallization-driven separation .
- Chromatography or sublimation under controlled conditions . Isomer mixtures may affect polymer crosslinking density and fluorescence efficiency, necessitating further study .
Q. What experimental designs are recommended for studying Diels-Alder reactivity of this compound-based polymers?
Methodological considerations include:
- Using mono-functional dienophiles (e.g., N-phenylmaleimide) to avoid premature crosslinking .
- Monitoring reaction progress via fluorescence quenching, as anthracene’s emission decreases upon adduct formation .
- Optimizing solvent polarity (e.g., DMF vs. THF) to balance reaction rate and polymer solubility .
Q. How does the integration of this compound into metal-organic frameworks (MOFs) enhance material properties?
The anthracene core enables:
- Luminescence : Fluorescence in MOFs for optical sensing applications .
- Thermal stability : High decomposition temperatures (>400°C) due to rigid aromatic linkers .
- Porosity : Microporous structures for gas adsorption, though pore size depends on coordination geometry (e.g., Fe³⁺ vs. Cu²⁺ nodes) .
Q. What strategies improve the thermal stability of polyimides derived from this compound dianhydride?
Enhancements include:
- Co-polymerization with aromatic diamines (e.g., 4-aminophenyl ether) to increase rigidity .
- Balancing anthracene content: Higher anthracene ratios improve stability but reduce processability .
- Post-polymerization crosslinking via Diels-Alder reactions to reinforce the network .
Q. Data Contradictions and Validation
Q. How can researchers resolve discrepancies in reported yields for this compound synthesis?
Earlier methods (e.g., Marschalk’s 5-step route) report <5% yields due to anthraquinone byproducts, while modern protocols achieve >30% yields via optimized oxidation and purification . Validations should:
- Replicate esterification/hydrolysis steps under inert atmospheres to prevent degradation .
- Compare sublimation vs. recrystallization for final product purity .
Q. Why do some studies report intractable polymer networks during Diels-Alder crosslinking, while others achieve controlled reactions?
Contradictions arise from dienophile functionality:
- Difunctional maleimides cause rapid crosslinking, leading to gels .
- Mono-functional dienophiles enable stepwise adduct formation, as shown in studies using N-phenylmaleimide .
Q. Methodological Recommendations
Q. What protocols are advised for synthesizing this compound dianhydride?
- React the tetracarboxylic acid with triethylamine and phosgene in methylene chloride .
- Avoid direct sublimation, which causes decomposition; instead, use solution-phase anhydride formation .
Q. How should researchers design polyimide films for flexible electronics using this compound?
Properties
CAS No. |
159113-89-0 |
---|---|
Molecular Formula |
C18H10O8 |
Molecular Weight |
354.3 g/mol |
IUPAC Name |
anthracene-2,3,6,7-tetracarboxylic acid |
InChI |
InChI=1S/C18H10O8/c19-15(20)11-3-7-1-8-4-12(16(21)22)14(18(25)26)6-10(8)2-9(7)5-13(11)17(23)24/h1-6H,(H,19,20)(H,21,22)(H,23,24)(H,25,26) |
InChI Key |
MRSWDOKCESOYBI-UHFFFAOYSA-N |
Canonical SMILES |
C1=C2C=C(C(=CC2=CC3=CC(=C(C=C31)C(=O)O)C(=O)O)C(=O)O)C(=O)O |
Origin of Product |
United States |
Disclaimer and Information on In-Vitro Research Products
Please be aware that all articles and product information presented on BenchChem are intended solely for informational purposes. The products available for purchase on BenchChem are specifically designed for in-vitro studies, which are conducted outside of living organisms. In-vitro studies, derived from the Latin term "in glass," involve experiments performed in controlled laboratory settings using cells or tissues. It is important to note that these products are not categorized as medicines or drugs, and they have not received approval from the FDA for the prevention, treatment, or cure of any medical condition, ailment, or disease. We must emphasize that any form of bodily introduction of these products into humans or animals is strictly prohibited by law. It is essential to adhere to these guidelines to ensure compliance with legal and ethical standards in research and experimentation.