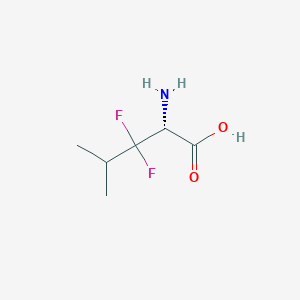
beta,beta-Difluoro leucine
- Click on QUICK INQUIRY to receive a quote from our team of experts.
- With the quality product at a COMPETITIVE price, you can focus more on your research.
Overview
Description
Beta,beta-Difluoro leucine is a fluorinated derivative of the essential amino acid leucine, where two fluorine atoms replace hydrogen atoms at the beta-carbon position. This structural modification enhances its metabolic stability and resistance to enzymatic degradation compared to non-fluorinated leucine, making it valuable in pharmaceutical and biochemical applications .
Preparation Methods
Synthetic Routes and Reaction Conditions
One common method is the fluorination of leucine derivatives using fluorinating agents such as bis(2-methoxyethyl)aminosulfur trifluoride (Deoxo-Fluor reagent) or aminodifluorosulfinium tetrafluoroborate salts . These reagents are effective for converting alcohols, aldehydes, and ketones into their corresponding gem-difluorides under mild reaction conditions .
Industrial Production Methods
Industrial production of 3,3-Difluoro-L-leucine may involve large-scale fluorination processes using similar reagents and conditions as described above. The choice of fluorinating agent and reaction conditions can be optimized to achieve high yields and purity of the desired product. Additionally, continuous exchange cell-free protein synthesis (CFPS) has been employed to produce fluorinated amino acids, including 3,3-Difluoro-L-leucine, for use in protein engineering and structural studies .
Chemical Reactions Analysis
Types of Reactions
3,3-Difluoro-L-leucine can undergo various chemical reactions, including:
Substitution Reactions: The fluorine atoms in 3,3-Difluoro-L-leucine can participate in nucleophilic substitution reactions, leading to the formation of new compounds.
Oxidation and Reduction Reactions: The compound can be oxidized or reduced under specific conditions to yield different products.
Hydrogenation Reactions: Asymmetric transfer hydrogenation of 3,3-difluoro-substituted compounds can be achieved using chiral phosphoric acid catalysts and Hantzsch ester as the hydrogen source.
Common Reagents and Conditions
Common reagents used in the reactions of 3,3-Difluoro-L-leucine include fluorinating agents (e.g., Deoxo-Fluor reagent), oxidizing agents, reducing agents, and hydrogenation catalysts. Reaction conditions such as temperature, solvent, and pH can be adjusted to optimize the yield and selectivity of the desired products .
Major Products Formed
The major products formed from the reactions of 3,3-Difluoro-L-leucine depend on the specific reaction conditions and reagents used. For example, nucleophilic substitution reactions can yield various fluorinated derivatives, while hydrogenation reactions can produce optically pure fluorinated compounds .
Scientific Research Applications
Structural Biology
Beta,beta-difluoro leucine is utilized in structural biology for the following reasons:
- Nuclear Magnetic Resonance Spectroscopy : The incorporation of this compound into proteins allows for the use of 19F nuclear magnetic resonance spectroscopy to study protein structure and dynamics. This technique provides insights into conformational changes and interactions within proteins, enhancing our understanding of their functions.
Medicinal Chemistry
In medicinal chemistry, this compound plays a crucial role in:
- Peptide Drug Design : Fluorinated amino acids like this compound are used to design peptide drugs with improved metabolic stability and bioavailability. The fluorination alters the lipophilicity and binding interactions, leading to enhanced therapeutic efficacy .
- Immunostimulatory Compounds : Analog compounds such as 3,3-difluoro-3,4-dideoxy-KRN7000 have demonstrated potent immunostimulatory effects, indicating potential applications in immunotherapy .
Protein Engineering
The use of this compound in protein engineering includes:
- Enhanced Stability : Incorporating this compound into enzymes can improve their stability and catalytic properties. This enhancement is critical for developing more efficient biocatalysts for industrial applications.
- Peptide Synthesis : Studies have shown that dipeptides containing this compound can be synthesized successfully, paving the way for creating complex peptide structures with desirable properties .
Analytical Chemistry
In analytical chemistry, this compound is significant for:
- High-Performance Liquid Chromatography Tandem Mass Spectrometry : Methods have been developed to determine d- and l-isomers of leucine, including this compound in biological samples. This capability is essential for studying metabolic pathways and understanding the pharmacokinetics of fluorinated amino acids.
Case Study 1: Stability of Difluoro-Oxytocin
A notable study demonstrated that this compound could be incorporated into oxytocin to create a difluoro variant with enhanced stability. The difluoro-oxytocin exhibited greater resistance to degradation compared to wild-type oxytocin when subjected to oxidative conditions, achieving a 63% yield post-folding. This stability is attributed to the unique properties imparted by the fluorinated amino acid .
Case Study 2: Fluorescent Conjugates
Research involving BODIPY-conjugated amino acids highlighted that this compound could be utilized in creating fluorescent probes for cellular imaging. These conjugates displayed selective affinity for specific cell types (e.g., Langerhans β-cells), indicating potential applications in biomedical research for tracking cellular processes and disease states .
Mechanism of Action
The mechanism of action of 3,3-Difluoro-L-leucine involves its incorporation into proteins and peptides, where it can influence their structure and function. The presence of fluorine atoms can alter the lipophilicity, metabolic stability, and binding interactions of the compound. In structural biology, 3,3-Difluoro-L-leucine can be used as a probe to study protein-ligand interactions and protein dynamics using 19F NMR spectroscopy .
Comparison with Similar Compounds
Structural and Functional Comparisons
The table below compares beta,beta-Difluoro leucine with structurally or functionally related compounds:
Compound | Structure/Modification | Key Biological Activity | Applications | Metabolic Stability |
---|---|---|---|---|
This compound | Fluorination at beta-carbon | Enhanced resistance to degradation; potential modulation of receptor/enzyme interactions | Drug design, enzyme inhibition | High |
HMB (Beta-hydroxy-beta-methylbutyrate) | Leucine metabolite with hydroxyl/methyl groups | Reduces muscle proteolysis, enhances muscle recovery | Sports nutrition, muscle preservation | Moderate |
Leucine derivative 26e (4-Piperidin-1-yl-phenyl amide) | Fluorinated leucine-based agonist | Potent β₃-adrenergic receptor agonist (EC₅₀: 0.008 µM) | Obesity/type 2 diabetes therapeutics | High |
L-Leucine beta-naphthylamide | Naphthylamide conjugation | Substrate for proteolytic enzymes (e.g., aminopeptidases) | Biochemical assays | Low |
Dihydroergocryptine | Hydrogenated ergot alkaloid with leucine substitution | Dopamine D₂ receptor agonist | Parkinson’s disease, migraine prophylaxis | Moderate |
Key Research Findings
- Metabolic Stability : Fluorination in this compound likely reduces susceptibility to oxidative deamination and transamination, similar to 4,4-difluoropiperidines, which exhibit prolonged half-lives .
- Receptor Interactions: Leucine derivative 26e demonstrates that fluorination enhances selectivity and potency for β₃-adrenergic receptors compared to non-fluorinated analogs, suggesting similar advantages for this compound in targeting leucine-sensitive pathways .
- Muscle Metabolism : Unlike HMB, which attenuates exercise-induced muscle damage via leucine catabolism, this compound’s fluorination may block its conversion to HMB, redirecting its role toward protein synthesis modulation .
- Enzymatic Assays: L-Leucine beta-naphthylamide is hydrolyzed by aminopeptidases, but fluorination at the beta position in this compound could inhibit such cleavage, as seen in fluorinated protease substrates .
Divergent Properties
- Toxicity Profile : While some fluorinated compounds (e.g., PFAS) raise environmental concerns, this compound’s smaller structure and lack of long alkyl chains may reduce bioaccumulation risks .
- Insulin Secretion: Leucine stimulates insulin secretion via mTOR activation, but this compound’s altered metabolism might decouple this effect, as seen in fluorinated analogs that bypass canonical pathways .
Future Research Directions
- Pharmacokinetic Studies: Compare oral bioavailability and tissue distribution with non-fluorinated leucine.
- Target Validation: Screen this compound against leucine-responsive receptors (e.g., mTOR, β₃-adrenergic receptors) to identify novel therapeutic niches.
- Toxicological Screening : Assess long-term safety, leveraging data from fluorinated agrochemicals and pharmaceuticals .
Biological Activity
Beta,beta-Difluoro leucine (β,β-DFL) is a fluorinated derivative of the essential amino acid leucine. Its unique structure, characterized by the substitution of two fluorine atoms at the beta carbon, significantly alters its physical and chemical properties, making it an intriguing subject in medicinal chemistry and biochemistry. This article explores the biological activity of β,β-DFL, focusing on its synthesis, interactions, and potential applications in drug design.
Chemical Structure and Properties
The chemical formula of β,β-Difluoro leucine is C6H10F2N2O2. The introduction of fluorine atoms enhances the stability and bioactivity of peptides and proteins, influencing their conformational dynamics. This modification can lead to improved interactions with biological targets, making β,β-DFL a valuable compound for further research.
Synthesis Methods
Synthesis of β,β-Difluoro leucine can be achieved through various methods, including:
- Enzymatic Hydrolysis : Utilizing specific enzymes to catalyze the hydrolysis of difluoro α-amino esters.
- Biomimetic Approaches : Employing biomimetic conditions for enantioselective synthesis, which allows for the rapid assembly of enantiomerically enriched difluoro amino acids from readily available starting materials .
- Fluorination Reactions : Using fluorinating agents under controlled conditions to achieve selective substitution at the beta position .
Interaction with Biological Systems
β,β-Difluoro leucine has been shown to interact with various biological systems effectively:
- Substrate for Enzymes : It acts as a substrate for human CCRF-CEM folypoly-γ-glutamate synthetase, demonstrating its potential role in metabolic pathways .
- Immunostimulatory Effects : Analog compounds like 3,3-difluoro-3,4-dideoxy-KRN7000 have been identified as potent immunostimulators .
- Peptide Stability : Studies have indicated that difluoro analogs exhibit higher stability compared to their non-fluorinated counterparts. For instance, difluoro-oxytocin synthesized from β,β-DFL showed improved stability under physiological conditions .
Case Study 1: Stability of Difluoro-Oxytocin
A study demonstrated that β,β-Difluoro leucine could be incorporated into oxytocin to create a difluoro variant with enhanced stability. The difluoro-oxytocin exhibited a higher resistance to degradation compared to wild-type oxytocin when subjected to oxidative conditions (63% yield post-folding)【5】.
Case Study 2: Fluorescent Conjugates
Research involving BODIPY-conjugated amino acids showed that β,β-Difluoro leucine could be utilized in creating fluorescent probes for cellular imaging. These conjugates displayed selective affinity for specific cell types (e.g., Langerhans β-cells), indicating potential applications in biomedical research【3】.
Comparative Analysis with Similar Compounds
The following table summarizes the structural similarities and unique features of β,β-Difluoro leucine compared to related compounds:
Compound | Structure Description | Unique Features |
---|---|---|
3,3-Difluoro-L-leucine | Similar structure but different stereochemistry | Distinct biological effects due to stereochemistry |
Beta-fluoro alanine | Contains only one fluorine atom | Less sterically hindered than β,β-Difluoro leucine |
3-Fluoroleucine | One fluorine substituent | Used in studies related to neurotransmitter activity |
3,4-Difluoroproline | Difluorination at different positions | Affects conformational properties significantly |
Q & A
Basic Research Questions
Q. What are the established synthetic routes for beta,beta-Difluoro leucine, and what analytical techniques are critical for confirming its purity and structure?
this compound is typically synthesized via fluorination of leucine precursors using reagents like diethylaminosulfur trifluoride (DAST) or Deoxo-Fluor. Key steps include protecting the amino and carboxyl groups to avoid side reactions during fluorination. Post-synthesis, purity is confirmed via 1H and 19F NMR to verify fluorine incorporation and absence of byproducts. High-resolution mass spectrometry (HRMS) and HPLC with UV/fluorescence detection are essential for structural validation. Experimental protocols must detail reaction conditions (temperature, solvent, stoichiometry) to ensure reproducibility .
Q. How does this compound interact with leucine-specific enzymes (e.g., leucyl-tRNA synthetase), and what experimental assays are used to study these interactions?
Competitive inhibition assays are commonly employed to assess enzyme binding. For example, kinetic parameters (Km, Vmax) are measured using spectrophotometric assays monitoring ATP consumption (coupled enzyme systems) or radiolabeled 14C-leucine displacement. X-ray crystallography or cryo-EM can resolve structural changes in enzymes upon binding. Studies should include controls with wild-type leucine to establish baseline activity and validate fluorinated analog effects .
Q. What metabolic pathways are disrupted by this compound in prokaryotic vs. eukaryotic systems, and how are these effects quantified?
Targeted metabolomics (LC-MS/MS) tracks incorporation into proteins or accumulation in metabolic intermediates. Isotope tracing with 13C-labeled analogs identifies perturbations in the TCA cycle or branched-chain amino acid catabolism. Comparative studies require parallel in vitro (bacterial cultures) and in vivo (murine models) setups, with dose-response curves to differentiate toxicity thresholds .
Advanced Research Questions
Q. What strategies are recommended for resolving contradictory data on the metabolic stability of this compound across different in vitro and in vivo models?
Cross-model validation involves replicating experiments in standardized cell lines (e.g., HEK293, HepG2) and primary cells under identical nutrient conditions. Discrepancies may arise from differential expression of fluorinated-amino-acid transporters or detoxification enzymes (e.g., glutathione transferases). Meta-analyses of proteomic datasets can identify conserved vs. context-dependent effects. Applying the FINER criteria (Feasible, Interesting, Novel, Ethical, Relevant) ensures hypotheses address mechanistic gaps rather than technical artifacts .
Q. How can isotopic labeling of this compound be optimized for tracking its distribution in cellular systems, and what analytical challenges must be addressed?
Dual 19F/13C labeling enables simultaneous tracking via NMR and MS. Challenges include isotopic dilution in nutrient-rich media and 19F signal quenching in dense tissues. Microfluidic systems with controlled nutrient delivery improve signal-to-noise ratios. Data should be normalized to intracellular fluorine concentration measured by ICP-MS to account for uptake variability .
Q. What computational models predict the conformational effects of this compound incorporation into proteins, and how do these align with empirical data?
Molecular dynamics (MD) simulations using force fields parameterized for fluorinated amino acids (e.g., CHARMM36m) predict steric and electronic impacts on protein folding. Empirical validation involves circular dichroism (CD) spectroscopy and thermal shift assays to compare mutant vs. wild-type protein stability. Discrepancies between simulation and experimental melting temperatures (Tm) may indicate incomplete force-field parameterization .
Q. Methodological Frameworks for Data Interpretation
Q. How should researchers design studies to distinguish between direct enzymatic inhibition and off-target metabolic effects of this compound?
- Step 1: Use CRISPR-engineered cell lines lacking specific transporters (e.g., LAT1) to isolate uptake-dependent effects.
- Step 2: Employ activity-based protein profiling (ABPP) with fluorophosphonate probes to map enzyme inhibition patterns.
- Step 3: Integrate transcriptomic data (RNA-seq) to identify compensatory pathways activated post-treatment.
- Validation: Compare results with leucine supplementation controls to rule out nutrient deprivation artifacts .
Q. What criteria should guide the selection of model organisms for in vivo studies of this compound toxicity?
Prioritize organisms with conserved leucine metabolism pathways (e.g., C. elegans for mitochondrial studies, zebrafish for developmental toxicity). Ethical considerations (3Rs framework) and genetic tractability (e.g., knockout models for bcat2, the branched-chain aminotransferase) are critical. Pilot studies must establish pharmacokinetic parameters (half-life, bioaccumulation) to justify sample sizes .
Q. Tables for Key Data Comparison
Properties
Molecular Formula |
C6H11F2NO2 |
---|---|
Molecular Weight |
167.15 g/mol |
IUPAC Name |
(2R)-2-amino-3,3-difluoro-4-methylpentanoic acid |
InChI |
InChI=1S/C6H11F2NO2/c1-3(2)6(7,8)4(9)5(10)11/h3-4H,9H2,1-2H3,(H,10,11)/t4-/m1/s1 |
InChI Key |
XXVSVJMOOGHOQD-SCSAIBSYSA-N |
Isomeric SMILES |
CC(C)C([C@@H](C(=O)O)N)(F)F |
Canonical SMILES |
CC(C)C(C(C(=O)O)N)(F)F |
Origin of Product |
United States |
Disclaimer and Information on In-Vitro Research Products
Please be aware that all articles and product information presented on BenchChem are intended solely for informational purposes. The products available for purchase on BenchChem are specifically designed for in-vitro studies, which are conducted outside of living organisms. In-vitro studies, derived from the Latin term "in glass," involve experiments performed in controlled laboratory settings using cells or tissues. It is important to note that these products are not categorized as medicines or drugs, and they have not received approval from the FDA for the prevention, treatment, or cure of any medical condition, ailment, or disease. We must emphasize that any form of bodily introduction of these products into humans or animals is strictly prohibited by law. It is essential to adhere to these guidelines to ensure compliance with legal and ethical standards in research and experimentation.