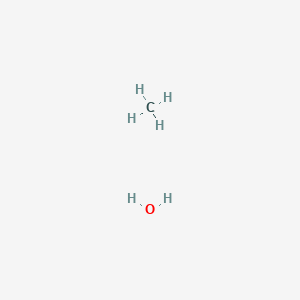
Carbon-water
- Click on QUICK INQUIRY to receive a quote from our team of experts.
- With the quality product at a COMPETITIVE price, you can focus more on your research.
Overview
Description
"Carbon-water" encompasses diverse contexts in chemistry and environmental science, including hydrogeochemical systems, organic pollutant analysis, and material interactions. This term broadly refers to:
- Inorganic this compound systems: Dissolved CO₂, carbonate (CO₃²⁻), and bicarbonate (HCO₃⁻) ions in water, typically studied in karst aquifers and carbonate-rich environments .
- Organic this compound systems: Total organic carbon (TOC) in water, which quantifies all dissolved or suspended organic pollutants .
- Material science interactions: Nonbonded forces between graphitic carbon (e.g., graphene, carbon nanotubes) and water molecules .
This article compares these this compound systems with analogous compounds or parameters, supported by data from recent studies.
Preparation Methods
Synthetic Routes and Reaction Conditions
Carbonic acid is typically formed through the dissolution of carbon dioxide in water: [ \text{CO}_2 + \text{H}_2\text{O} \rightarrow \text{H}_2\text{CO}_3 ]
This reaction occurs naturally in the environment, especially in bodies of water where carbon dioxide from the atmosphere dissolves. In laboratory settings, carbonic acid can be prepared by bubbling carbon dioxide gas through water under controlled conditions.
Industrial Production Methods
In industrial settings, carbonic acid is not typically produced in large quantities due to its instability. Instead, its derivatives, such as sodium bicarbonate (NaHCO₃) and calcium carbonate (CaCO₃), are produced on a large scale. These compounds are used in various applications, including baking, pharmaceuticals, and construction.
Chemical Reactions Analysis
Fundamental Reaction of CO₂ with Water
The most well-studied reaction involves carbon dioxide (CO₂) reacting with water to form carbonic acid (H₂CO₃) , which dissociates into bicarbonate (HCO₃⁻) and protons (H⁺) :
CO2(aq)+H2O⇌H++HCO3−
This equilibrium governs pH changes in aqueous systems, such as in ocean acidification and biological respiration experiments .
Key Observations:
-
Reversibility : Heating the solution shifts the equilibrium to the left, driving off CO₂ and restoring the original pH .
-
Indicator Response : pH-sensitive indicators like phenol red (yellow to red at pH 6.8–8.4) or thymolphthalein (blue to colorless at pH 9.3–10.5) visualize this reaction .
Reaction with Alkaline Solutions
CO₂ reacts with sodium hydroxide (NaOH) to form sodium carbonate (Na₂CO₃) :
2NaOH(aq)+CO2(g)→Na2CO3(aq)+H2O(l)
This reaction is foundational in carbon capture and storage (CCS) technologies, where alkaline solutions facilitate CO₂ absorption .
Mineral Carbonation and Ocean Acidification
In natural systems, CO₂ reacts with calcium ions to form calcium carbonate (CaCO₃) :
CO32−+Ca2+→CaCO3(s)
This process is critical for:
-
Ocean acidification : Excess CO₂ reduces carbonate ion availability (CO₃²⁻), impairing marine calcification .
-
Carbon sequestration : The net reaction in seawater is:
CO2+H2O+CO32−⇌2HCO3−
This consumes carbonate ions, lowering pH and destabilizing marine ecosystems .
Advanced Reaction Pathways Under Extreme Conditions
Recent studies reveal complex reactions in nanoconfined and supercritical water environments:
Nanoconfinement Effects
-
Enhanced reactivity : CO₂ in nanoconfined spaces reacts more vigorously, forming intermediates like pyrocarbonate (C₂O₅²⁻) , previously considered unstable in bulk water .
-
Interfacial chemistry : Solid-liquid interfaces (e.g., graphene or stishovite) alter acidity and reaction mechanisms, shifting equilibria .
Supercritical Water Dynamics
-
Markov state models : First-principles simulations show CO₂ hydrolysis involves collective proton transfer along water chains, with stepwise mechanisms under nanoconfinement .
-
Pyrocarbonate stability : The anion [C₂O₅²⁻] persists in confined solutions due to superionic behavior, challenging prior assumptions about its transient nature .
Time-Scale Dynamics
Asymptotic analysis of CO₂ dissolution reveals:
-
Rapid CO₂ dissolution (seconds).
-
Slow bicarbonate-to-carbonate conversion (minutes to hours).
Equilibrium Constants
Reaction | Equilibrium Constant (25°C) | Source |
---|---|---|
CO₂ + H₂O ↔ H⁺ + HCO₃⁻ | K1≈4.3×10−7 | |
HCO₃⁻ ↔ H⁺ + CO₃²⁻ | K2≈4.8×10−11 | |
Ca²⁺ + CO₃²⁻ ↔ CaCO₃(s) | Ksp≈4.5×10−9 |
Implications for Carbon Cycle and Technology
-
Deep Earth processes : Nanoconfined CO₂-water reactions suggest enhanced mineral carbonation in Earth’s mantle, influencing long-term carbon storage .
-
CCS optimization : Understanding pyrocarbonate formation and proton transfer mechanisms could improve CO₂ sequestration efficiency .
-
Ocean chemistry : The CO₃²⁻/HCO₃⁻ equilibrium directly impacts marine biodiversity and climate regulation .
Experimental Validation
-
Classroom demonstrations : Breathing into indicator solutions (e.g., phenol red) visually confirms CO₂-mediated acidification .
-
Advanced simulations : Ab initio molecular dynamics and Markov models validate theoretical predictions for complex systems .
Research Frontiers
-
Unexplored intermediates : Further identification of oxocarbons (e.g., C₃O₆²⁻) in aqueous systems.
-
Pressure-temperature effects : High-pressure simulations to replicate deep-Earth conditions .
-
Technological adaptation : Leveraging nanoconfined systems for industrial CO₂ mineralization.
This synthesis integrates foundational and cutting-edge research, emphasizing the dynamic interplay between CO₂, water, and environmental conditions. The findings highlight the multifaceted role of carbon-water reactions in Earth’s carbon cycle and human-driven systems.
Scientific Research Applications
Water Treatment
Activated carbon plays a crucial role in water treatment processes, effectively removing contaminants from drinking water, wastewater, and industrial effluents. The primary applications include:
- Drinking Water Treatment : Activated carbon is used to adsorb organic compounds, chlorine, and other impurities from municipal water supplies. Granulated activated carbon systems are commonly employed for this purpose due to their efficiency in dechlorination and removal of taste and odor-causing substances .
- Wastewater Treatment : In wastewater management, activated carbon is utilized to remove volatile organic compounds and other pollutants. It is particularly effective in treating industrial wastewater where specific contaminants must be eliminated to meet regulatory standards .
- Groundwater Remediation : Activated carbon can be used to remediate contaminated groundwater by adsorbing harmful substances. This method is often employed in environmental cleanup projects to restore polluted aquifers .
Case Study: Activated Carbon in Municipal Water Treatment
A municipal water treatment facility implemented a granular activated carbon system that resulted in a 90% reduction in organic contaminants over a six-month period. This not only improved water quality but also reduced the need for chemical coagulants, leading to cost savings.
Air Purification
Activated carbon is extensively used in air purification systems due to its high surface area and porosity, which allow it to capture airborne pollutants effectively. Applications include:
- Indoor Air Quality Improvement : Activated carbon filters are utilized in residential and commercial buildings to remove odors, smoke, and volatile organic compounds from indoor air .
- Industrial Emission Control : Industries use activated carbon to capture harmful emissions before they are released into the atmosphere, thus complying with environmental regulations .
Medical Applications
In the medical field, activated carbon is recognized for its ability to adsorb toxins and drugs:
- Poisoning Treatment : Activated carbon is administered in cases of poisoning or overdose as it binds to toxic substances in the gastrointestinal tract, preventing their absorption into the bloodstream .
- Analytical Chemistry : Activated carbon is used as a stationary phase in chromatographic techniques for separating various compounds in biological samples .
Carbon Dioxide Utilization
Carbon dioxide can be dissolved in water to form carbonic acid, which has applications in water treatment:
- pH Adjustment : Carbon dioxide is used to lower the pH of wastewater without introducing additional salts that could disrupt biological processes. This method is safer and more environmentally friendly compared to traditional strong acids .
Case Study: CO2 for pH Control in Wastewater Treatment
A wastewater treatment plant adopted carbon dioxide for pH adjustment instead of sulfuric acid. This change led to reduced corrosion of equipment and lower operational costs while maintaining compliance with discharge regulations.
Environmental Applications
Activated carbon's role extends beyond water treatment into broader environmental applications:
- Pollutant Capture : Activated carbon is effective in capturing pollutants from air and water streams during industrial processes . It has been successfully used to mitigate environmental damage from spills and leaks.
- Sustainable Practices : Biomass-derived activated carbons have shown promise in removing pharmaceuticals from wastewater, contributing to sustainable waste management practices .
Data Table: Summary of Applications
Application Area | Specific Use Cases | Effectiveness |
---|---|---|
Water Treatment | Drinking water filtration | 90% reduction in organic contaminants |
Wastewater Treatment | Industrial effluent treatment | Significant removal of VOCs |
Air Purification | Indoor air quality improvement | Effective removal of odors and pollutants |
Medical | Poisoning treatment | Binds toxins preventing absorption |
Environmental Cleanup | Groundwater remediation | Restores contaminated aquifers |
pH Adjustment | Wastewater treatment | Safer than strong acids; reduces corrosion |
Mechanism of Action
Carbonic acid exerts its effects through its ability to donate and accept protons (H⁺ ions). This property allows it to participate in acid-base reactions and buffering systems. In biological systems, carbonic acid is involved in the reversible hydration of carbon dioxide, catalyzed by the enzyme carbonic anhydrase: [ \text{CO}_2 + \text{H}_2\text{O} \xleftrightarrow{\text{carbonic anhydrase}} \text{H}_2\text{CO}_3 ]
Comparison with Similar Compounds
Inorganic Carbon-Water vs. Other Mineral-Rich Waters
Carbonate waters (dominated by HCO₃⁻/CO₃²⁻) differ from sulfate- or chloride-dominated waters in geochemical behavior:
Key Findings :
- Carbonate waters buffer pH effectively, reducing heavy metal toxicity compared to sulfate-rich acidic waters .
- Ionic strength in carbonate systems correlates with PCO₂ (partial pressure of CO₂), influencing mineral saturation indices .
TOC vs. Other Water Quality Parameters (COD, BOD)
TOC measures total organic carbon, while COD (chemical oxygen demand) and BOD (biochemical oxygen demand) assess oxidizable pollutants:
Key Findings :
- TOC is the most comprehensive for organic pollution but cannot replace COD/BOD in assessing biodegradability .
- COD/BOD correlations are critical for modeling wastewater treatment kinetics .
Graphitic this compound Interactions vs. Other Nanomaterial-Water Systems
Graphitic carbon’s hydrophobic/hydrophilic behavior contrasts with silica or TiO₂ nanoparticles:
Key Findings :
- Graphitic carbon’s interaction energy aligns with experimental CNT radial breathing mode (RBM) frequency shifts .
- Silica’s high hydrophilicity stems from strong hydrogen bonding, unlike graphite’s basal plane .
Research Advancements and Challenges
- Carbonate Waters : Machine learning (ML) and remote sensing (RS) improve large-scale this compound flux monitoring but require high-quality data .
- TOC Analysis : Combustion-infrared methods achieve 98% accuracy but struggle with volatile organic compounds .
- Graphitic Carbon : Ab initio models (e.g., MP2, CCSD(T)) predict interfacial properties but face scalability issues .
Biological Activity
The biological activity of carbon-water, particularly in the context of activated carbon and its interactions with water, is a significant area of research in environmental science and engineering. This article explores the biological activity associated with carbon materials, particularly activated carbon, and their applications in water treatment processes.
Overview of Activated Carbon
Activated carbon (AC) is a porous material derived from carbonaceous sources such as coal, wood, or coconut shells. Its high surface area and porous structure make it an effective adsorbent for various organic and inorganic contaminants in water. The biological activity of activated carbon is enhanced when it is used in conjunction with biological processes, leading to the development of Biological Activated Carbon (BAC) systems.
Mechanisms of Biological Activity
- Adsorption : Activated carbon adsorbs organic compounds from water, which can then serve as a substrate for microbial growth. This process enhances the degradation of pollutants through microbial metabolism.
- Biofilm Formation : Microorganisms attach to the surface of activated carbon, forming biofilms that facilitate the breakdown of organic matter. The efficiency of this process depends on factors such as contact time, flow rates, and the characteristics of the activated carbon used.
- Nutrient Cycling : The presence of microorganisms on activated carbon can lead to improved nutrient cycling within aquatic systems, contributing to overall water quality improvement.
1. Biological Activated Carbon in Golf Course Ponds
A study evaluated the effectiveness of BAC systems in improving the water quality of golf course ponds. Two ponds were monitored: one using BAC treatment and a reference pond without treatment.
Parameter | Pond A (BAC) | Pond B (Reference) |
---|---|---|
Total Phosphorus (TP) | Decreased significantly | Stable |
Chemical Oxygen Demand (COD) | Reduced by 40% | No significant change |
Attached Biomass Concentration | Increased over time | Low levels |
The results indicated that BAC effectively reduced TP and COD levels in treated ponds compared to untreated ones, demonstrating the potential for BAC systems in managing nutrient pollution in stagnant water bodies .
2. Removal of Pesticides from Water
Research focused on developing activated carbon specifically designed for pesticide removal from water highlighted the importance of surface functional groups in enhancing adsorption capabilities. The study found that:
- Carbon Source : Activated carbons derived from starch showed superior performance due to their mesoporous structure.
- Functional Groups : The presence of hydroxyl and carbonyl groups significantly increased pesticide adsorption efficiency.
This case exemplifies how tailored activated carbon can enhance biological activity by providing optimal conditions for microbial degradation while simultaneously removing harmful contaminants .
Research Findings
Recent studies have provided insights into various aspects of the biological activity associated with this compound systems:
- Ozonation-Biofiltration Processes : A study demonstrated that ozonation followed by biofiltration using activated carbon could effectively reduce phenolic compounds in water. The optimal ozonation dose was identified as 1.64 mg O(3)/mg TOC, with contact times ranging from 2.4 to 24 minutes showing varying degrees of effectiveness .
- Toxicological Assessments : Research has also highlighted potential toxic effects associated with hydraulic fracturing wastewater when treated with activated carbon systems. These findings emphasize the need for careful evaluation of both the benefits and potential risks associated with using AC in environmental applications .
Q & A
Basic Research Questions
Q. How do researchers experimentally quantify carbon-water interactions in terrestrial ecosystems?
Methodological Answer: Utilize remote sensing data combined with diagnostic models (e.g., PML-V2) to estimate evapotranspiration and carbon fluxes. Statistical methods like partial differential attribution analysis isolate variables (e.g., vegetation impact on water cycles) . Basin-scale observations and remote sensing datasets validate model outputs, ensuring spatial-temporal accuracy .
Q. What experimental designs are effective for isolating this compound feedback mechanisms in field studies?
Methodological Answer: Implement controlled scenario simulations (e.g., vegetation manipulation) and paired watershed studies. Use high-frequency sensors to monitor soil moisture, CO₂ fluxes, and microclimate variables. Statistical controls (e.g., ANOVA) differentiate natural variability from treatment effects .
Q. How can contradictory findings in this compound flux data be resolved?
Methodological Answer: Apply sensitivity analysis to identify model parameter uncertainties. Cross-validate results using multi-model ensembles (e.g., combining PML-V2 with hydrological models) and ground-truth data from eddy covariance towers .
Q. What are standard protocols for measuring water-use efficiency (WUE) in plants?
Methodological Answer: Calculate WUE as the ratio of net CO₂ assimilation to transpiration using gas exchange chambers. For ecosystem-scale WUE, integrate eddy covariance data with remote sensing-derived evapotranspiration .
Q. How do researchers address data gaps in long-term this compound cycle studies?
Methodological Answer: Leverage historical satellite datasets (e.g., MODIS, Landsat) and reanalysis products (e.g., ERA5). Gap-filling algorithms (e.g., machine learning imputation) reconstruct missing data while quantifying uncertainty .
Advanced Research Questions
Q. What computational models best capture the non-linear dynamics of this compound coupling in arid ecosystems?
Methodological Answer: Develop digital twin ecosystems using AI-driven parallel intelligence systems. These integrate real-time data (e.g., soil carbon, precipitation) with mechanistic models to simulate feedbacks at plant, community, and socio-economic levels .
Q. How can multi-scale observations (leaf to ecosystem) refine this compound coupling theories?
Methodological Answer: Deploy nested sensor networks (e.g., leaf-level porometers, canopy lidar) and harmonize data via flux upscaling algorithms. Coupled indicator systems (e.g., WUE, carbon sequestration rate) bridge scales .
Q. What mechanistic insights do litter-soil interface studies provide on this compound synergies?
Methodological Answer: Track litter decomposition kinetics using isotopic tracers (e.g., ¹³C) and measure water-holding capacity shifts via gravimetric analysis. Meta-analyses of global litter datasets reveal soil carbon stabilization pathways .
Q. How do low-carbon policies influence regional water resource allocation models?
Methodological Answer: Integrate life-cycle assessment (LCA) of carbon footprints into water allocation algorithms. System network models optimize trade-offs between carbon sinks (e.g., afforestation) and water security in basins like the Haihe .
Q. What emerging technologies enhance the resolution of this compound flux measurements?
Methodological Answer: Deploy quantum cascade laser spectrometers for real-time δ¹³C-CO₂ and H₂O isotopologue tracking. Nanomaterials (e.g., sub-2-nm carbon nanotubes) enable precise pore-scale transport studies .
Q. Methodological Trends & Challenges
Q. What bibliometric tools identify research gaps in this compound studies?
Methodological Answer: Use CiteSpace for co-citation analysis of Web of Science datasets. Keyword clustering (e.g., "eco-hydrological resilience") highlights understudied areas like socio-economic drivers .
Q. How can AI improve uncertainty quantification in this compound models?
Methodological Answer: Train convolutional neural networks (CNNs) on heterogeneous datasets (e.g., soil maps, climate projections) to predict confidence intervals. Explainable AI (XAI) methods like SHAP values decode black-box predictions .
Q. What experimental frameworks assess climate feedbacks on this compound symbiosis?
Methodological Answer: Conduct factorial experiments combining elevated CO₂, warming, and drought treatments. Process-based models (e.g., CLM) simulate interactions, validated via flux tower networks .
Properties
CAS No. |
154320-69-1 |
---|---|
Molecular Formula |
CH6O |
Molecular Weight |
34.058 g/mol |
IUPAC Name |
methane;hydrate |
InChI |
InChI=1S/CH4.H2O/h1H4;1H2 |
InChI Key |
VUZPPFZMUPKLLV-UHFFFAOYSA-N |
Canonical SMILES |
C.O |
Origin of Product |
United States |
Disclaimer and Information on In-Vitro Research Products
Please be aware that all articles and product information presented on BenchChem are intended solely for informational purposes. The products available for purchase on BenchChem are specifically designed for in-vitro studies, which are conducted outside of living organisms. In-vitro studies, derived from the Latin term "in glass," involve experiments performed in controlled laboratory settings using cells or tissues. It is important to note that these products are not categorized as medicines or drugs, and they have not received approval from the FDA for the prevention, treatment, or cure of any medical condition, ailment, or disease. We must emphasize that any form of bodily introduction of these products into humans or animals is strictly prohibited by law. It is essential to adhere to these guidelines to ensure compliance with legal and ethical standards in research and experimentation.