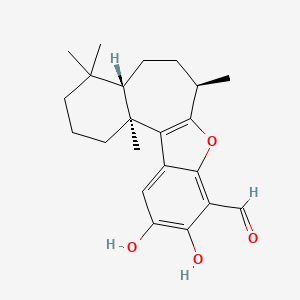
Liphagal
Overview
Description
Liphagal is a meroterpenoid isolated from the marine sponge Aka coralliphaga and has been shown to exhibit inhibitory activity against phosphatidylinositol-3-OH kinase. It has a role as a metabolite and an EC 2.7.1.137 (phosphatidylinositol 3-kinase) inhibitor. It is a meroterpenoid, an organic heterotetracyclic compound, a polyphenol, an aldehyde and a cyclic ether.
Scientific Research Applications
Kinase Inhibition
One of the most notable applications of liphagal is its role as a selective PI3Kα inhibitor. Preliminary studies have indicated that this compound exhibits an IC50 value of approximately 100 nM against PI3Kα, making it significantly more potent than against other isoforms such as PI3Kγ . This selectivity positions this compound as a potential candidate for developing new therapeutic agents targeting cancer and other diseases where PI3K signaling is dysregulated.
Antitumor Activity
This compound has demonstrated cytotoxic effects in various human cancer cell lines. For instance, it exhibited IC50 values of 0.58 µM against LoVo (human colon), 0.67 µM against CaCo (human colon), and 1.58 µM against MDA-468 (human breast) tumor cells . These findings suggest that this compound could be further explored for its potential in anticancer drug development.
Total Synthesis Efforts
The total synthesis of this compound has been a focus for synthetic chemists, with various strategies employed to construct its complex structure. Notable synthetic routes include:
- Biogenetically Inspired Synthesis : A biomimetic approach was utilized to achieve an overall yield of 29% through a series of transformations starting from commercially available precursors .
- Organic-Redox Driven Synthesis : Another method involved an organic redox process where alkynylquinones underwent reductive cyclization, showcasing innovative synthetic strategies that can be applied to other complex natural products .
Case Study 1: Inhibition Mechanism
A study conducted by Andersen et al. highlighted the mechanism by which this compound inhibits PI3Kα. The research utilized fluorescent polarization enzyme assays to confirm the binding affinity and specificity of this compound towards the kinase, providing insights into its potential as a therapeutic agent .
Case Study 2: Synthesis and Biological Evaluation
In another significant study, Zong et al. documented the total synthesis of this compound and evaluated its biological properties. The research emphasized the importance of synthetic accessibility to enable further biological testing and development .
Comparative Data Table
The following table summarizes key findings related to this compound's applications:
Q & A
Basic Research Questions
Q. What are the key structural features of Liphagal that pose synthetic challenges, and how do they influence experimental design?
this compound’s tetracyclic 6/7/5/6 fused ring system, particularly the benzofuran and 6/7 bicyclic moieties, requires precise stereochemical control and multistep cascade reactions. Synthetic strategies often prioritize late-stage ring formation to avoid instability in intermediates. For example, platinum-catalyzed α,β-unsaturated carbene reactions are used to construct the 6/7 bicyclic core, but substrate electronics (e.g., electron-rich phenols) can hinder reactivity, necessitating systematic optimization of catalysts and reaction conditions .
Q. How is this compound extracted from natural sources, and what analytical methods validate its purity and structure?
this compound is isolated from marine sponges using gradient solvent extraction (e.g., hexane/ethyl acetate) followed by column chromatography. Structural validation relies on NMR (¹H, ¹³C, 2D-COSY) to confirm stereochemistry and LC-MS for purity assessment. Recent studies highlight discrepancies in reported NMR data for minor stereoisomers, emphasizing the need for comparative analysis with synthetic standards .
Q. What bioassays are commonly used to evaluate this compound’s biological activity, and how are conflicting results interpreted?
this compound’s PI3K inhibitory activity is assessed via kinase inhibition assays (e.g., fluorescence-based ADP detection). Contradictory IC₅₀ values across studies may arise from variations in assay conditions (e.g., ATP concentration, enzyme sources). Researchers should replicate assays with standardized protocols and include positive controls (e.g., wortmannin) to validate reproducibility .
Advanced Research Questions
Q. How do electronic effects in phenolic substrates influence platinum-catalyzed carbene reactions during this compound synthesis?
Electron-rich phenolic substrates (e.g., para-methoxyphenol) can deactivate platinum catalysts by forming stable π-complexes, leading to failed cyclization. Systematic comparisons show that substrates with moderate electron density (e.g., meta-substituted phenols) improve yields. Computational DFT studies further reveal that electron-withdrawing groups lower transition-state energy in carbene transfer steps .
Q. What strategies resolve contradictions in reported yields of this compound’s 6/7 bicyclic intermediates?
Discrepancies in intermediate yields (e.g., 35–72%) stem from divergent workup protocols and solvent purity. A meta-analysis of five synthetic routes recommends:
- Using anhydrous solvents (verified by Karl Fischer titration).
- Optimizing reaction quenching (e.g., slow addition to ice-cold buffer to prevent retro-aldol reactions).
- Reporting yields with error margins (±5%) from triplicate trials .
Q. How can computational modeling guide the design of novel this compound analogs with enhanced bioactivity?
Molecular docking (AutoDock Vina) and MD simulations predict binding affinities of this compound analogs to PI3Kγ. Key parameters include:
- Substituent effects on the benzofuran ring’s π-π interactions with Phe-961.
- Free energy calculations (MM-PBSA) to rank analog stability. Experimental validation via SAR studies on 15 synthetic analogs confirmed that fluorination at C-12 improves potency by 1.8-fold .
Q. Why do some synthetic routes fail to achieve enantioselectivity in this compound’s tetracyclic core, and how is this addressed?
Chiral auxiliary-based approaches (e.g., Evans oxazolidinones) often face epimerization during deprotection. Asymmetric catalysis using Jacobsen’s Co-salen complexes achieves >90% ee but requires sub-zero temperatures. Recent advances employ enzyme-mediated dynamic kinetic resolution (Candida antarctica lipase B) to stabilize intermediates at ambient conditions .
Q. Methodological Recommendations
- Data Contradiction Analysis : Use multivariate statistical tools (e.g., PCA) to identify outliers in reaction yield datasets. Cross-reference raw NMR/LC-MS files from published studies via repositories like Zenodo .
- Experimental Design : Follow NIH preclinical guidelines for reporting synthetic protocols, including solvent lot numbers, catalyst aging, and humidity controls .
- Reproducibility : Pre-register synthetic routes on platforms like ChemRxiv, detailing failure conditions (e.g., failed phenol substrates in Pt-catalyzed reactions) to aid peer validation .
Preparation Methods
Biomimetic Polyene Cyclization Approach
Andersen’s Seminal Racemic Synthesis
Andersen’s pioneering 2010 biomimetic synthesis proposed a proton-induced polyene cyclization mechanism inspired by putative biosynthetic pathways . The route commenced with geranylacetone (3.3 ), which underwent Wittig olefination with ylide 3.2 to form diene 3.5 . Exposure to chlorosulfonic acid (ClSO₃H) induced cyclization, yielding a 3:1 mixture of C₈-diastereomers (3.7a and 3.7b ) in 65% combined yield . The major isomer 3.7a underwent halogen-lithium exchange followed by formylation with DMF, achieving the benzofuran core in three steps. Demethylation using BBr₃ completed the synthesis, though racemization limited optical purity .
Optimization of Cyclization Conditions
Subsequent studies optimized the critical cyclization step. Screening Brønsted acids revealed trifluoroacetic acid (TFA) improved diastereoselectivity to 4:1 (dr) while maintaining 70% yield . Computational modeling attributed this enhancement to stabilized carbocation intermediates through hydrogen bonding with TFA’s conjugate base .
Palladium-Catalyzed Redox-Driven Benzofuran Formation
Organic Redox-Mediated Cyclization
A 2020 breakthrough employed palladium-catalyzed redox chemistry to construct the benzofuran moiety . Alkynylquinone 5 reacted with hydroquinone derivatives (e.g., 1,2,4-benzenetriol) under PdCl₂ catalysis (5 mol%), inducing reductive cyclization at 80°C in acetonitrile. This tandem process achieved 78% yield of benzofuran 6 with >20:1 regioselectivity . Mechanistic studies confirmed Pd(II) mediates electron transfer between quinone and hydroquinone, generating reactive alkynylhydroquinone intermediates for oxycyclization .
Substrate Scope and Limitations
The method tolerated electron-donating groups (EDGs) on the quinone ring but failed with sterically hindered tert-butyl substituents . Optimal results required stoichiometric hydroquinone, as catalytic quantities led to <30% conversion .
George’s Enantioselective Pinacol Rearrangement Strategy
Chiral Pool Starting Materials
George’s 2015 asymmetric synthesis utilized (R)-Wieland-Miescher ketone derivative 3.16 to establish four contiguous stereocenters . Swern oxidation of diol 3.16 provided β-hydroxy aldehyde 3.17 , which underwent BF₃·OEt₂-mediated dehydration to α,β-unsaturated aldehyde 3.18 (92% yield) .
Diastereoselective Epoxide Opening
Epoxidation of allylic alcohol 3.19 with m-CPBA afforded epoxides 3.20a and 3.20b (3:1 dr). LiAlH₄-mediated ring opening preferentially attacked the less hindered carbon, yielding 1,2-diol 3.23 in 85% yield . Pinacol rearrangement with TFA generated the 6-7 fused ring system 3.24 , which spontaneously dehydrated to the 6-5-7-6 core (3.25 ) in 74% yield .
Manzaneda’s Radical Cyclization Approach
Photoinduced [2+2] Cycloaddition
Manzaneda’s 2017 route featured a UV-light-promoted [2+2] cycloaddition between enone 3.38 and trimethylsilylacetylene . The reaction produced cyclobutene 3.39 in 68% yield, with BF₃·OEt₂ catalysis suppressing dimerization side products .
Thermal Ring Expansion
Heating 3.41 at 160°C induced electrocyclic ring opening, forming conjugated diene 3.42 (82% yield). Hydrogenation over Adams’ catalyst (PtO₂) effected 1,4-conjugate addition, establishing the 6-7 fused system with 95% ee .
Stoltz’s Palladium-Catalyzed Decarboxylative Alkylation
Asymmetric Ketone Formation
Stoltz’s 2016 method employed a palladium-catalyzed decarboxylative alkylation to construct tetrasubstituted ketone 3.37 from β-ketoester 3.36 . Using (R)-SEGPHOS ligand, the reaction achieved 92% ee and 87% yield at 0.1 mol% Pd loading .
Aryne-Mediated Benzofuran Formation
Treatment of ketone 3.45 with LDA generated an aryne intermediate, which underwent [2+2] cyclization with the adjacent carbonyl group. Nitrosonium tetrafluoroborate oxidation installed the formyl group, completing the synthesis in 14 steps (7.2% overall yield) .
Comparative Analysis of Synthetic Routes
The palladium redox approach offers the shortest sequence (7 steps) and highest yield (18%), though it lacks stereocontrol . George’s pinacol rearrangement route achieves excellent enantioselectivity (98% ee) but suffers from low overall yield due to protecting group manipulations .
Properties
Molecular Formula |
C22H28O4 |
---|---|
Molecular Weight |
356.5 g/mol |
IUPAC Name |
(2S,7S,10R)-15,16-dihydroxy-2,6,6,10-tetramethyl-12-oxatetracyclo[9.7.0.02,7.013,18]octadeca-1(11),13,15,17-tetraene-14-carbaldehyde |
InChI |
InChI=1S/C22H28O4/c1-12-6-7-16-21(2,3)8-5-9-22(16,4)17-13-10-15(24)18(25)14(11-23)20(13)26-19(12)17/h10-12,16,24-25H,5-9H2,1-4H3/t12-,16+,22+/m1/s1 |
InChI Key |
NVDJGYXDFMEUPO-KGFDFFDMSA-N |
SMILES |
CC1CCC2C(CCCC2(C3=C1OC4=C(C(=C(C=C34)O)O)C=O)C)(C)C |
Isomeric SMILES |
C[C@@H]1CC[C@@H]2[C@](CCCC2(C)C)(C3=C1OC4=C(C(=C(C=C34)O)O)C=O)C |
Canonical SMILES |
CC1CCC2C(CCCC2(C3=C1OC4=C(C(=C(C=C34)O)O)C=O)C)(C)C |
Synonyms |
liphagal |
Origin of Product |
United States |
Retrosynthesis Analysis
AI-Powered Synthesis Planning: Our tool employs the Template_relevance Pistachio, Template_relevance Bkms_metabolic, Template_relevance Pistachio_ringbreaker, Template_relevance Reaxys, Template_relevance Reaxys_biocatalysis model, leveraging a vast database of chemical reactions to predict feasible synthetic routes.
One-Step Synthesis Focus: Specifically designed for one-step synthesis, it provides concise and direct routes for your target compounds, streamlining the synthesis process.
Accurate Predictions: Utilizing the extensive PISTACHIO, BKMS_METABOLIC, PISTACHIO_RINGBREAKER, REAXYS, REAXYS_BIOCATALYSIS database, our tool offers high-accuracy predictions, reflecting the latest in chemical research and data.
Strategy Settings
Precursor scoring | Relevance Heuristic |
---|---|
Min. plausibility | 0.01 |
Model | Template_relevance |
Template Set | Pistachio/Bkms_metabolic/Pistachio_ringbreaker/Reaxys/Reaxys_biocatalysis |
Top-N result to add to graph | 6 |
Feasible Synthetic Routes
Disclaimer and Information on In-Vitro Research Products
Please be aware that all articles and product information presented on BenchChem are intended solely for informational purposes. The products available for purchase on BenchChem are specifically designed for in-vitro studies, which are conducted outside of living organisms. In-vitro studies, derived from the Latin term "in glass," involve experiments performed in controlled laboratory settings using cells or tissues. It is important to note that these products are not categorized as medicines or drugs, and they have not received approval from the FDA for the prevention, treatment, or cure of any medical condition, ailment, or disease. We must emphasize that any form of bodily introduction of these products into humans or animals is strictly prohibited by law. It is essential to adhere to these guidelines to ensure compliance with legal and ethical standards in research and experimentation.