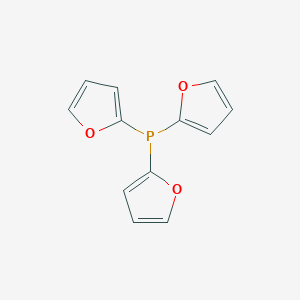
Tri(2-furyl)phosphine
Overview
Description
Tri(2-furyl)phosphine (P(C₄H₃O)₃) is a tertiary phosphine ligand featuring three 2-furyl substituents. Its molecular formula is C₁₂H₉O₃P, with a molar mass of 232.17 g/mol and a melting point of 62°C . This ligand is characterized by its low steric demand and π-accepting properties, making it versatile in transition-metal catalysis, particularly in cross-coupling reactions such as Negishi and Stille couplings . Its heteroaromatic furyl groups contribute to unique electronic and steric profiles, distinguishing it from conventional alkyl- or arylphosphines.
Preparation Methods
Grignard Reagent-Mediated Synthesis of Tri(2-furyl)phosphine
The most well-documented method for synthesizing this compound involves a Grignard reagent-based approach, as detailed in the Chinese patent CN103012480A . This method employs phosphorus trichloride (PCl<sub>3</sub>) and furan-derived Grignard reagents under controlled conditions to achieve high purity and yield.
Reaction Mechanism and Stepwise Procedure
The synthesis proceeds via a three-step sequence:
-
Formation of the Furylmagnesium Bromide Grignard Reagent :
Furan reacts with magnesium turnings in tetrahydrofuran (THF) at 60–65°C for 2 hours to generate the furylmagnesium bromide intermediate. This step requires strict anhydrous conditions to prevent hydrolysis of the Grignard reagent. -
Nucleophilic Substitution with PCl<sub>3</sub> :
The furylmagnesium bromide is cooled to -10–0°C, and PCl<sub>3</sub> is added dropwise. The reaction mixture is stirred for an additional hour, facilitating the substitution of chlorine atoms in PCl<sub>3</sub> with furyl groups. -
Hydrolysis and Purification :
The intermediate is quenched with aqueous ammonium chloride (NH<sub>4</sub>Cl) at 0–5°C, followed by extraction with dichloromethane. The crude product is purified via recrystallization from ethanol, yielding this compound as a white crystalline solid .
Optimization and Yield Data
Key parameters influencing the reaction efficiency include temperature control, solvent selection, and stoichiometry. The patent reports a yield of 85–88.5% with a purity exceeding 99% after recrystallization . The table below summarizes the critical reaction conditions:
Step | Reagents/Conditions | Temperature | Time | Yield/Purity |
---|---|---|---|---|
Grignard formation | Mg, THF, furan | 60–65°C | 2 h | - |
PCl<sub>3</sub> addition | Furylmagnesium bromide, PCl<sub>3</sub> | -10–0°C | 1 h | - |
Hydrolysis | NH<sub>4</sub>Cl, H<sub>2</sub>O | 0–5°C | - | 85–88.5% |
Recrystallization | Ethanol | Ambient | - | >99% purity |
This method avoids highly toxic reagents like PH<sub>3</sub> and employs mild conditions, making it suitable for industrial-scale production .
Alternative Synthetic Routes and Comparative Analysis
While the Grignard approach dominates current methodologies, other phosphine syntheses provide contextual insights:
PH<sub>3</sub>-Based Nucleophilic Addition (For Ethyl-Spaced Analogues)
Artem’ev et al. synthesized tris[2-(2-furyl)ethyl]phosphine—a structural analogue—by reacting PH<sub>3</sub> with 2-vinylfuran in a KOH/DMSO superbase system . Although this method achieves 65% yield, it requires handling toxic PH<sub>3</sub> gas and specialized equipment, limiting its practicality for PFu<sub>3</sub> synthesis .
Challenges in Direct Furyl Substitution
Unlike arylphosphines, direct substitution of PCl<sub>3</sub> with furan derivatives faces steric and electronic hurdles due to furan’s aromatic instability. The Grignard method circumvents this by leveraging the strong nucleophilicity of furylmagnesium bromide .
Applications in Coordination Chemistry
This compound’s utility is exemplified in its reactions with transition metal clusters. For instance, PFu<sub>3</sub> reacts with triosmium carbonyl clusters to form complexes featuring furyne and phosphinidene ligands, demonstrating its role in facilitating C–H and P–C bond activation . These applications underscore the importance of high-purity PFu<sub>3</sub> for advanced material synthesis.
Chemical Reactions Analysis
Substitution Reactions with Manganese Carbonyl Complexes
PFu<sub>3</sub> reacts with labile manganese carbonyl precursors to form substituted derivatives. Key findings include:
Reaction with [Mn<sub>2</sub>(CO)<sub>9</sub>(NCMe)]
-
Product : [Mn<sub>2</sub>(CO)<sub>9</sub>(PFu<sub>3</sub>)] (1)
-
Conditions : Room temperature in CH<sub>2</sub>Cl<sub>2</sub>, 24 hours.
-
Characterization :
Reaction with [Mn<sub>2</sub>(CO)<sub>8</sub>(NCMe)<sub>2</sub>]
-
Products :
-
[Mn<sub>2</sub>(CO)<sub>9</sub>(PFu<sub>3</sub>)] (1) (6% yield)
-
[Mn<sub>2</sub>(CO)<sub>8</sub>(PFu<sub>3</sub>)<sub>2</sub>] (2) (15% yield)
-
-
Conditions : Room temperature in CH<sub>2</sub>Cl<sub>2</sub>, 24 hours .
-
Characterization of 2 :
Phosphorus–Carbon Bond Cleavage Reactions
Thermal treatment of PFu<sub>3</sub> with Mn<sub>2</sub>(CO)<sub>10</sub> induces bond cleavage, yielding mononuclear complexes:
Reaction with Mn<sub>2</sub>(CO)<sub>10</sub>
-
Products :
-
[Mn<sub>2</sub>(CO)<sub>8</sub>(PFu<sub>3</sub>)<sub>2</sub>] (2) (29% yield)
-
mer-[Mn(CO)<sub>3</sub>(η<sup>1</sup>-C<sub>4</sub>H<sub>3</sub>O)(PFu<sub>3</sub>)<sub>2</sub>] (3) (18% yield)
-
-
Structural Analysis of 3 :
Bond/Parameter | Value |
---|---|
Mn–P distances | 2.2594(7) Å, 2.2597(7) Å |
Mn–C (furyl) distance | 2.050(2) Å |
Trans OC–Mn–CO angle | 169.76(10)° |
-
Coordination Geometry : Distorted octahedral with meridional CO ligands and mutually trans PFu<sub>3</sub> groups .
Comparative Reactivity with Other Phosphines
PFu<sub>3</sub> exhibits electronic differences compared to PPh<sub>3</sub>, despite similar Tolman cone angles (PFu<sub>3</sub> = 133°; PPh<sub>3</sub> = 145°). Notable contrasts include:
Property | PFu<sub>3</sub> | PPh<sub>3</sub> |
---|---|---|
Electron-withdrawing effect | Higher due to furyl rings | Lower (phenyl groups) |
Catalytic activity | Enhanced in cross-coupling | Moderate |
This electronic profile enables PFu<sub>3</sub> to stabilize low-oxidation-state metal centers more effectively .
Mechanistic Insights
-
Ligand Substitution : Proceeds via dissociative pathways, facilitated by labile acetonitrile (NCMe) ligands in precursors.
-
Bond Cleavage : Heating induces Mn–Mn bond cleavage in Mn<sub>2</sub>(CO)<sub>10</sub>, followed by P–C bond scission in PFu<sub>3</sub>, generating η<sup>1</sup>-furyl ligands .
Scientific Research Applications
Catalytic Applications
1.1 Coordination Chemistry
TFP is primarily employed as a ligand in coordination complexes, particularly with transition metals. Its ability to donate electrons through its aromatic furan rings enhances its reactivity compared to traditional phosphines like triphenylphosphine (PPh₃). Notably, TFP exhibits a lower Tolman cone angle (133°) than PPh₃ (145°), indicating a more compact structure that influences its interaction with metal centers in catalysis .
Table 1: Comparison of Ligand Properties
Ligand | Tolman Cone Angle | Reactivity |
---|---|---|
This compound | 133° | High |
Triphenylphosphine | 145° | Moderate |
Tri(2-thienyl)phosphine | 135° | Moderate |
1.2 Palladium-Catalyzed Reactions
TFP has been extensively studied in palladium-catalyzed cross-coupling reactions, including the Sonogashira reaction, which forms carbon-carbon bonds between alkynes and aryl halides. Research shows that TFP-palladium complexes outperform traditional PPh₃-based catalysts in terms of efficiency and yield .
Case Study: Sonogashira Coupling
- Conditions: TFP (20 mol%) was used alongside Pd(OAc)₂ in toluene at 110 °C for 18 hours.
- Results: The reaction yielded high selectivity and conversion rates, demonstrating TFP's effectiveness as a ligand .
Organic Synthesis
2.1 Staudinger Reduction
TFP serves as a nucleophile in the Staudinger reduction, converting azides to amines. This transformation highlights TFP's versatility beyond coordination chemistry and showcases its role in organic synthesis methodologies.
Table 2: Summary of Organic Transformations Using TFP
Reaction Type | Role of TFP | Outcome |
---|---|---|
Staudinger Reduction | Nucleophile | Conversion of azides to amines |
Cross-Coupling | Ligand | Formation of C-C bonds |
Reactivity Studies
Recent studies have explored the reactivity of TFP with various transition metal complexes. For instance, reactions with manganese carbonyl complexes have been documented, revealing how TFP can stabilize metal centers and facilitate complex formation.
Case Study: Manganese Complexes
Mechanism of Action
The mechanism by which tri(2-furyl)phosphine exerts its effects is primarily through its role as a ligand. It coordinates with transition metals, forming complexes that facilitate various chemical reactions. The furan rings provide electron-donating properties, enhancing the stability and reactivity of the metal complexes. This coordination can influence the electronic properties of the metal center, thereby affecting the overall reaction pathway .
Comparison with Similar Compounds
Electronic Properties
Tri(2-furyl)phosphine exhibits moderate σ-donating strength and notable π-accepting ability due to the electron-withdrawing nature of the furyl oxygen atoms. This contrasts with:
- Tri(1-adamantyl)phosphine: Exceptionally strong σ-donor (higher electron-releasing character) due to bulky adamantyl groups, enhancing catalytic activity in Suzuki-Miyaura couplings .
- Triphenylphosphine (PPh₃): Weaker π-acceptor but widely used due to balanced σ-donation and commercial availability. In palladium-catalyzed aminocarbonylation, PPh₃ yielded 76% product vs. 66% for this compound .
- Tri(2-thienyl)phosphine: Similar π-accepting capacity but slightly stronger σ-donor due to sulfur's polarizability. In enantioselective allylation, Tri(2-thienyl)phosphine provided higher enantioselectivity (87% ee) but lower yield (65%) compared to this compound (82% yield, 81% ee) .
Steric Effects
The low steric bulk of this compound facilitates the formation of reactive metal complexes. For example:
- In palladium-catalyzed reactions, its compact structure enables efficient oxidative addition, outperforming bulky ligands like tris(2,6-dimethoxyphenyl)phosphine in coupling reactions .
- Comparatively, Tri(1-adamantyl)phosphine 's steric hindrance stabilizes metal centers against decomposition but limits substrate accessibility .
Catalytic Performance
- Negishi Coupling: this compound is critical for coupling brominated pyridazinones, achieving high yields where PPh₃ fails .
- Stille Reactions : Ligand efficiency follows AsPh₃ > this compound > PPh₃ , with this compound enabling faster polymerization of thiophene-phenylene copolymers .
- Enantioselective Synthesis : Cooperates with dinuclear zinc catalysts, though less effective than TPPO in diynylation reactions (68% ee vs. TPPO's 90% ee) .
Stability and Reactivity
- Gold Complexes : Forms stable 1:1 complexes (e.g., L-Au-Cl) without heterocycle-metal interactions. Dinuclear species [(LAu)₂X]⁺ exhibit high stability in mass spectrometry .
- Orthometallation : Under reflux, reacts with osmium clusters to form metallated derivatives, indicating reactivity under harsh conditions .
Biological Activity
Tri(2-furyl)phosphine, often denoted as PFu3, is a phosphine compound characterized by three furyl groups attached to a phosphorus atom. This compound has garnered attention in various fields, particularly in catalysis and medicinal chemistry, due to its unique electronic properties and biological activities. This article delves into the biological activity of this compound, highlighting its anticancer properties, reactivity with transition metals, and potential applications in drug development.
Anticancer Activity
Recent studies have demonstrated that this compound exhibits significant cytotoxic effects against various cancer cell lines. Notably, a study involving iron(II) cyclopentadienyl complexes revealed that the complex containing this compound (designated as complex 2b) showed cytotoxicity against HL-60 (human promyelocytic leukemia) and A549 (non-small cell lung cancer) cells. The half-maximal inhibitory concentration (IC50) values were reported as 10.03 µM for HL-60 cells and 73.54 µM for A549 cells, indicating a stronger effect on the leukemia cell line compared to lung cancer cells .
Table 1: Cytotoxicity of Iron(II) Complexes Containing this compound
Complex | Cell Line | IC50 (µM) |
---|---|---|
2b | HL-60 | 10.03 |
2b | A549 | 73.54 |
The genotoxic potential of complex 2b was also assessed, revealing that it does not induce strand breaks in double-stranded DNA, suggesting a different mechanism of action compared to other more genotoxic complexes .
Reactivity with Transition Metals
This compound has been extensively studied for its reactivity with various transition metal complexes. For instance, reactions with manganese carbonyl complexes have been documented, where PFu3 facilitated the formation of substituted products through phosphorus-carbon bond cleavage . This reactivity is attributed to the unique electronic properties of this compound, which differ from those of traditional phosphines like triphenylphosphine.
Table 2: Reactivity of this compound with Transition Metal Complexes
Metal Complex | Reaction Conditions | Product(s) |
---|---|---|
[Mn2(CO)10] | Room temperature | [Mn2(CO)9(PFu3)] |
[Ru4(μ-H)4(CO)12] | Refluxing THF | Substituted products |
The ability of this compound to stabilize metal centers while facilitating catalytic transformations makes it a valuable ligand in organometallic chemistry.
Case Studies and Research Findings
Several case studies have illustrated the biological activity and potential applications of this compound:
- Anticancer Drug Development : The incorporation of this compound into iron complexes has shown promise as an anticancer agent, particularly against leukemia cells. The research indicates potential pathways for developing new therapeutic agents targeting specific cancer types .
- Catalysis : Studies have shown that transition metal complexes containing this compound exhibit enhanced catalytic activity compared to those with traditional phosphines. This is particularly evident in reactions involving nitrile hydration and carbon-carbon bond formation .
- Structural Studies : X-ray crystallography has been employed to elucidate the structures of metal complexes formed with this compound, providing insights into the bonding interactions that contribute to their biological activity .
Q & A
Basic Research Questions
Q. What are the primary synthetic routes for Tri(2-furyl)phosphine, and how can its purity be validated?
this compound is typically synthesized via nucleophilic substitution reactions between phosphorus precursors (e.g., PCl₃) and 2-furyl Grignard reagents. Post-synthesis, purity is validated using nuclear magnetic resonance (¹H, ¹³C, and ³¹P NMR) to confirm the absence of unreacted starting materials or byproducts. Mass spectrometry (MS) further corroborates molecular weight integrity. For structural confirmation, single-crystal X-ray diffraction is recommended, as demonstrated in ruthenium cluster studies .
Q. How is this compound characterized spectroscopically, and what key spectral markers distinguish it from analogous ligands?
Key spectroscopic markers include:
- ³¹P NMR : A singlet near δ 20–25 ppm, indicative of a monodentate phosphine ligand.
- ¹H NMR : Distinct furyl proton resonances at δ 6.3–7.5 ppm (coupled with phosphorus, J₃₁P–H ≈ 2–5 Hz).
- IR : C–O–C stretching vibrations (≈ 1250 cm⁻¹) from the furyl rings. These markers differentiate it from triphenylphosphine (³¹P NMR δ ~ -5 ppm) and other arylphosphines .
Q. What are the standard applications of this compound in catalysis?
It serves as a ligand in transition-metal-catalyzed reactions, particularly where steric and electronic tuning is critical. For example, in rhodium-catalyzed hydrogenative aldol couplings, it enables syn-diastereoselectivity >95% by moderating electron density at the metal center, unlike triphenylphosphine, which yields mixtures .
Advanced Research Questions
Q. How do the electronic properties of this compound influence its performance in asymmetric catalysis compared to triphenylphosphine?
The electron-withdrawing furyl groups reduce the ligand’s σ-donor strength, lowering electron density at the metal center. This enhances electrophilicity of intermediates, improving selectivity in reactions like Negishi coupling. For instance, replacing PPh₃ with this compound in palladium-catalyzed cross-couplings increases yields from <50% to >80% due to reduced catalyst poisoning .
Q. What structural insights into this compound-metal complexes have been revealed by X-ray crystallography?
X-ray studies of [Ru₂(CO)₆(μ-PFu₂)(μ-1,2-Fu)] complexes show:
- Ru–P bond lengths of 2.299–2.321 Å, shorter than Ru–PPh₃ bonds (≈2.35–2.40 Å), reflecting stronger π-backbonding.
- A μ-1,2-furyl bridging mode, enabling unique electron delocalization across the Ru₂ core. These features stabilize 34-electron clusters, critical for catalytic cycles in CO activation .
Q. How does this compound address contradictions in literature reports on ligand efficiency?
Discrepancies in catalytic outcomes (e.g., turnover frequency vs. selectivity) arise from solvent and counterion effects. Methodologically, controlled studies in anhydrous THF with [Rh(cod)₂]OTf show that this compound outperforms PPh₃ in diastereoselectivity (syn:anti ratio >20:1) due to its smaller cone angle (≈145° vs. 160° for PPh₃), reducing steric hindrance during substrate binding .
Q. What experimental strategies mitigate decomposition of this compound under oxidative conditions?
Degradation via furyl ring oxidation is minimized by:
- Using inert atmospheres (N₂/Ar) during reactions.
- Incorporating chelating auxiliaries (e.g., bidentate ligands) to stabilize the metal-phosphine adduct.
- Monitoring via ³¹P NMR to detect phosphine oxide formation (δ ≈ 25–30 ppm) .
Q. Methodological Tables
Table 1. Comparative Ligand Properties
Ligand | Cone Angle (°) | ³¹P NMR (δ, ppm) | Ru–P Bond Length (Å) |
---|---|---|---|
This compound | 145 | 20–25 | 2.299–2.321 |
PPh₃ | 160 | -5 to -10 | 2.35–2.40 |
P(2-furyl)₃ | 145 | 20–25 | 2.30–2.32 |
Data from . |
Table 2. Optimization of Aldol Coupling Conditions
Ligand | Solvent | Diastereoselectivity (syn:anti) | Yield (%) |
---|---|---|---|
This compound | THF | 20:1 | 85 |
PPh₃ | THF | 3:1 | 60 |
None | THF | <1:1 | 10 |
Conditions: [Rh(cod)₂]OTf (5 mol%), H₂ (1 atm), 25°C . |
Properties
IUPAC Name |
tris(furan-2-yl)phosphane | |
---|---|---|
Source | PubChem | |
URL | https://pubchem.ncbi.nlm.nih.gov | |
Description | Data deposited in or computed by PubChem | |
InChI |
InChI=1S/C12H9O3P/c1-4-10(13-7-1)16(11-5-2-8-14-11)12-6-3-9-15-12/h1-9H | |
Source | PubChem | |
URL | https://pubchem.ncbi.nlm.nih.gov | |
Description | Data deposited in or computed by PubChem | |
InChI Key |
DLQYXUGCCKQSRJ-UHFFFAOYSA-N | |
Source | PubChem | |
URL | https://pubchem.ncbi.nlm.nih.gov | |
Description | Data deposited in or computed by PubChem | |
Canonical SMILES |
C1=COC(=C1)P(C2=CC=CO2)C3=CC=CO3 | |
Source | PubChem | |
URL | https://pubchem.ncbi.nlm.nih.gov | |
Description | Data deposited in or computed by PubChem | |
Molecular Formula |
C12H9O3P | |
Source | PubChem | |
URL | https://pubchem.ncbi.nlm.nih.gov | |
Description | Data deposited in or computed by PubChem | |
DSSTOX Substance ID |
DTXSID00334690 | |
Record name | Tri(2-furyl)phosphine | |
Source | EPA DSSTox | |
URL | https://comptox.epa.gov/dashboard/DTXSID00334690 | |
Description | DSSTox provides a high quality public chemistry resource for supporting improved predictive toxicology. | |
Molecular Weight |
232.17 g/mol | |
Source | PubChem | |
URL | https://pubchem.ncbi.nlm.nih.gov | |
Description | Data deposited in or computed by PubChem | |
CAS No. |
5518-52-5 | |
Record name | Tri(2-furyl)phosphine | |
Source | EPA DSSTox | |
URL | https://comptox.epa.gov/dashboard/DTXSID00334690 | |
Description | DSSTox provides a high quality public chemistry resource for supporting improved predictive toxicology. | |
Record name | tris(furan-2-yl)phosphane | |
Source | European Chemicals Agency (ECHA) | |
URL | https://echa.europa.eu/information-on-chemicals | |
Description | The European Chemicals Agency (ECHA) is an agency of the European Union which is the driving force among regulatory authorities in implementing the EU's groundbreaking chemicals legislation for the benefit of human health and the environment as well as for innovation and competitiveness. | |
Explanation | Use of the information, documents and data from the ECHA website is subject to the terms and conditions of this Legal Notice, and subject to other binding limitations provided for under applicable law, the information, documents and data made available on the ECHA website may be reproduced, distributed and/or used, totally or in part, for non-commercial purposes provided that ECHA is acknowledged as the source: "Source: European Chemicals Agency, http://echa.europa.eu/". Such acknowledgement must be included in each copy of the material. ECHA permits and encourages organisations and individuals to create links to the ECHA website under the following cumulative conditions: Links can only be made to webpages that provide a link to the Legal Notice page. | |
Synthesis routes and methods
Procedure details
Retrosynthesis Analysis
AI-Powered Synthesis Planning: Our tool employs the Template_relevance Pistachio, Template_relevance Bkms_metabolic, Template_relevance Pistachio_ringbreaker, Template_relevance Reaxys, Template_relevance Reaxys_biocatalysis model, leveraging a vast database of chemical reactions to predict feasible synthetic routes.
One-Step Synthesis Focus: Specifically designed for one-step synthesis, it provides concise and direct routes for your target compounds, streamlining the synthesis process.
Accurate Predictions: Utilizing the extensive PISTACHIO, BKMS_METABOLIC, PISTACHIO_RINGBREAKER, REAXYS, REAXYS_BIOCATALYSIS database, our tool offers high-accuracy predictions, reflecting the latest in chemical research and data.
Strategy Settings
Precursor scoring | Relevance Heuristic |
---|---|
Min. plausibility | 0.01 |
Model | Template_relevance |
Template Set | Pistachio/Bkms_metabolic/Pistachio_ringbreaker/Reaxys/Reaxys_biocatalysis |
Top-N result to add to graph | 6 |
Feasible Synthetic Routes
Disclaimer and Information on In-Vitro Research Products
Please be aware that all articles and product information presented on BenchChem are intended solely for informational purposes. The products available for purchase on BenchChem are specifically designed for in-vitro studies, which are conducted outside of living organisms. In-vitro studies, derived from the Latin term "in glass," involve experiments performed in controlled laboratory settings using cells or tissues. It is important to note that these products are not categorized as medicines or drugs, and they have not received approval from the FDA for the prevention, treatment, or cure of any medical condition, ailment, or disease. We must emphasize that any form of bodily introduction of these products into humans or animals is strictly prohibited by law. It is essential to adhere to these guidelines to ensure compliance with legal and ethical standards in research and experimentation.