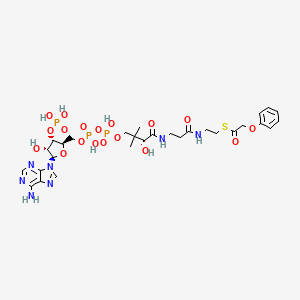
Phenoxyacetyl-CoA
- Click on QUICK INQUIRY to receive a quote from our team of experts.
- With the quality product at a COMPETITIVE price, you can focus more on your research.
Overview
Description
Phenoxyacetyl-CoA is an acyl-CoA that results from the formal condensation of the thiol group of coenzyme A with the carboxy group of phenoxyacetic acid. It derives from a phenoxyacetic acid.
Scientific Research Applications
Biosynthesis of Antibiotics
Penicillin Production
Phenoxyacetyl-CoA serves as a critical precursor in the biosynthetic pathway of penicillins. Specifically, it is involved in the acylation of 6-aminopenicillanic acid (6-APA) to produce various penicillin derivatives. The enzyme acyl-CoA:6-APA acyltransferase catalyzes this reaction, where this compound acts as the acyl donor. Research has shown that modifications to the this compound structure can lead to the synthesis of novel penicillin derivatives with enhanced antibacterial properties .
Enzymatic Mechanisms
Studies have identified specific enzymes responsible for activating this compound, highlighting its role in antibiotic biosynthesis. For instance, the phenylacetyl-CoA ligase gene from Penicillium chrysogenum has been cloned and characterized, demonstrating its importance in increasing penicillin production through genetic amplification . The disruption of this gene significantly reduces both this compound ligase activity and penicillin yield, illustrating the compound's crucial role in antibiotic synthesis .
Therapeutic Applications
Antibacterial Activity
this compound derivatives exhibit notable antibacterial properties. Research indicates that compounds derived from this compound can inhibit bacterial growth effectively, making them potential candidates for developing new antibiotics . The ability to modify the acyl chain length and structure allows for tailored antibacterial activity against resistant strains.
Cancer Treatment
Recent studies have explored the cytotoxic effects of this compound derivatives on various cancer cell lines. For example, certain modifications have shown promise in targeting specific cancer types by inducing apoptosis in malignant cells while sparing normal tissues . This suggests potential therapeutic applications beyond antibiotic use, particularly in oncology.
Biotechnological Innovations
Synthetic Biology
The integration of this compound into synthetic biology frameworks has opened avenues for engineering microbial strains capable of producing high-value pharmaceuticals. By manipulating metabolic pathways to enhance the flux towards this compound, researchers can optimize the production of desired compounds, including advanced antibiotics and anticancer agents .
Nanotechnology Applications
this compound has also found applications in nanotechnology, particularly in developing nanoparticles for drug delivery systems. Its chemical properties allow for the functionalization of nanoparticles, enhancing their stability and bioavailability while enabling targeted delivery mechanisms for therapeutic agents .
Case Studies and Research Findings
Q & A
Basic Research Questions
Q. What are the primary metabolic pathways involving Phenoxyacetyl-CoA, and how can researchers identify its role in cellular processes?
this compound participates in pathways such as odd-chain fatty acid biosynthesis and methylmalonyl-CoA metabolism. Researchers can map its role using isotopic tracing (e.g., ¹³C-labeled glucose) and analyze intermediates via LC-MS. Key enzymatic steps, such as pyruvate decarboxylation and succinyl-CoA isomerization, highlight its connection to propionyl-CoA pools . Pathway reconstruction in microbial systems (e.g., E. coli) with gene knockout studies can further validate its metabolic contributions .
Q. What methodological approaches are recommended for quantifying this compound concentrations in biological samples?
Use high-performance liquid chromatography coupled with tandem mass spectrometry (HPLC-MS/MS) for high sensitivity. Sample preparation must avoid enzymatic degradation by flash-freezing tissues and using protease inhibitors. Cross-contamination is minimized by changing pipette tips between replicates, and plate-based assays require strict adherence to incubation times and temperature controls .
Q. What analytical techniques effectively distinguish this compound from structural analogs like 4-Chlorothis compound?
Reverse-phase chromatography with UV detection (e.g., 254 nm) separates analogs based on hydrophobicity. Confirm identity via fragmentation patterns in MS/MS or NMR spectroscopy. Structural differences, such as chlorine substitution, alter retention times and spectral signatures .
Q. How should researchers design control experiments to validate the specificity of this compound detection assays?
Include negative controls (e.g., enzyme-free reactions or CoA-depleted samples) and spike recovery tests with pure this compound standards. Validate assay specificity using competitive inhibitors or CRISPR-edited cell lines lacking key biosynthetic enzymes .
Q. What are the structural determinants of this compound stability, and how should storage conditions be adjusted?
The thioester bond is prone to hydrolysis. Store lyophilized samples at -80°C in anhydrous conditions. In solution, maintain pH 6–7 and avoid repeated freeze-thaw cycles. Stabilizing agents like dithiothreitol (DTT) prevent oxidation .
Advanced Research Questions
Q. How can isotopic labeling techniques optimize the tracing of this compound flux in engineered microbial systems?
Use ¹³C-glucose to track carbon flow through glycolysis and the TCA cycle. Mass isotopomer distribution analysis (MIDA) quantifies labeling in downstream products like odd-chain fatty acids. Computational flux balance analysis (FBA) models reconcile experimental data with pathway stoichiometry .
Q. What strategies resolve contradictions between in vitro and in vivo activity data of this compound-dependent enzymes?
Discrepancies may arise from cellular compartmentalization or cofactor availability (e.g., NADPH/NADP+ ratios). Conduct in vivo kinetic assays using Förster resonance energy transfer (FRET) biosensors and compare with in vitro data. Adjust reaction conditions to mimic cytoplasmic redox states .
Q. How do variations in cofactor availability influence this compound biosynthesis yields in heterologous systems?
NADPH-dependent steps (e.g., pyruvate dehydrogenase) are bottlenecks. Overexpress NADPH-generating enzymes (e.g., glucose-6-phosphate dehydrogenase) or engineer NADH-dependent isoforms. Real-time monitoring with fluorescent cofactor probes (e.g., iNAP sensors) optimizes pathway flux .
Q. What computational modeling approaches best predict the kinetic parameters of this compound synthase under varying pH conditions?
Molecular dynamics (MD) simulations assess enzyme conformational changes at different pH levels. Michaelis-Menten parameters (Km, Vmax) derived from in silico docking (e.g., AutoDock) guide experimental validation. Machine learning models trained on homologous enzymes improve prediction accuracy .
Q. What are the implications of competing shunt pathways for efficient channeling of this compound in synthetic biology applications?
Competing pathways (e.g., β-oxidation or citrate synthase) reduce product yields. Use dynamic regulation (e.g., CRISPRi knockdowns) to suppress shunt enzymes. Metabolite scaffolding with engineered protein complexes (e.g., synthetic metabolons) enhances pathway efficiency .
Q. Methodological Notes
- Data Integrity : Adhere to ALCOA principles (Attributable, Legible, Contemporaneous, Original, Accurate) for reproducible results. Use electronic lab notebooks (ELNs) with timestamped entries .
- Contradiction Analysis : Compare datasets using Bland-Altman plots or hierarchical clustering to identify systemic biases. Replicate experiments under orthogonal conditions (e.g., aerobic vs. anaerobic) .
Properties
Molecular Formula |
C29H42N7O18P3S |
---|---|
Molecular Weight |
901.7 g/mol |
IUPAC Name |
S-[2-[3-[[(2R)-4-[[[(2R,3S,4R,5R)-5-(6-aminopurin-9-yl)-4-hydroxy-3-phosphonooxyoxolan-2-yl]methoxy-hydroxyphosphoryl]oxy-hydroxyphosphoryl]oxy-2-hydroxy-3,3-dimethylbutanoyl]amino]propanoylamino]ethyl] 2-phenoxyethanethioate |
InChI |
InChI=1S/C29H42N7O18P3S/c1-29(2,24(40)27(41)32-9-8-19(37)31-10-11-58-20(38)13-49-17-6-4-3-5-7-17)14-51-57(47,48)54-56(45,46)50-12-18-23(53-55(42,43)44)22(39)28(52-18)36-16-35-21-25(30)33-15-34-26(21)36/h3-7,15-16,18,22-24,28,39-40H,8-14H2,1-2H3,(H,31,37)(H,32,41)(H,45,46)(H,47,48)(H2,30,33,34)(H2,42,43,44)/t18-,22-,23-,24+,28-/m1/s1 |
InChI Key |
SBPBPUFWNOBPMN-CECATXLMSA-N |
SMILES |
CC(C)(COP(=O)(O)OP(=O)(O)OCC1C(C(C(O1)N2C=NC3=C(N=CN=C32)N)O)OP(=O)(O)O)C(C(=O)NCCC(=O)NCCSC(=O)COC4=CC=CC=C4)O |
Isomeric SMILES |
CC(C)(COP(=O)(O)OP(=O)(O)OC[C@@H]1[C@H]([C@H]([C@@H](O1)N2C=NC3=C(N=CN=C32)N)O)OP(=O)(O)O)[C@H](C(=O)NCCC(=O)NCCSC(=O)COC4=CC=CC=C4)O |
Canonical SMILES |
CC(C)(COP(=O)(O)OP(=O)(O)OCC1C(C(C(O1)N2C=NC3=C(N=CN=C32)N)O)OP(=O)(O)O)C(C(=O)NCCC(=O)NCCSC(=O)COC4=CC=CC=C4)O |
Origin of Product |
United States |
Retrosynthesis Analysis
AI-Powered Synthesis Planning: Our tool employs the Template_relevance Pistachio, Template_relevance Bkms_metabolic, Template_relevance Pistachio_ringbreaker, Template_relevance Reaxys, Template_relevance Reaxys_biocatalysis model, leveraging a vast database of chemical reactions to predict feasible synthetic routes.
One-Step Synthesis Focus: Specifically designed for one-step synthesis, it provides concise and direct routes for your target compounds, streamlining the synthesis process.
Accurate Predictions: Utilizing the extensive PISTACHIO, BKMS_METABOLIC, PISTACHIO_RINGBREAKER, REAXYS, REAXYS_BIOCATALYSIS database, our tool offers high-accuracy predictions, reflecting the latest in chemical research and data.
Strategy Settings
Precursor scoring | Relevance Heuristic |
---|---|
Min. plausibility | 0.01 |
Model | Template_relevance |
Template Set | Pistachio/Bkms_metabolic/Pistachio_ringbreaker/Reaxys/Reaxys_biocatalysis |
Top-N result to add to graph | 6 |
Feasible Synthetic Routes
Disclaimer and Information on In-Vitro Research Products
Please be aware that all articles and product information presented on BenchChem are intended solely for informational purposes. The products available for purchase on BenchChem are specifically designed for in-vitro studies, which are conducted outside of living organisms. In-vitro studies, derived from the Latin term "in glass," involve experiments performed in controlled laboratory settings using cells or tissues. It is important to note that these products are not categorized as medicines or drugs, and they have not received approval from the FDA for the prevention, treatment, or cure of any medical condition, ailment, or disease. We must emphasize that any form of bodily introduction of these products into humans or animals is strictly prohibited by law. It is essential to adhere to these guidelines to ensure compliance with legal and ethical standards in research and experimentation.