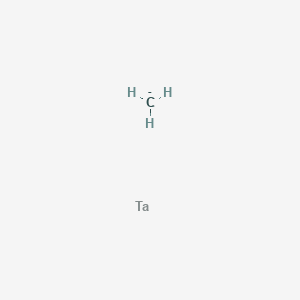
Tantalum(1+) methanide
- Click on QUICK INQUIRY to receive a quote from our team of experts.
- With the quality product at a COMPETITIVE price, you can focus more on your research.
Overview
Description
Tantalum(1+) methanide (chemical formula: TaCH₃) is a rare organometallic compound where tantalum exhibits a +1 oxidation state bonded to a methanide (CH₃⁻) ligand. This compound is of significant interest in materials science due to tantalum's unique properties, including its high melting point (3,017°C), corrosion resistance, and ability to form stable bonds with carbon-based ligands . While tantalum(V) oxide (Ta₂O₅) and other high-oxidation-state compounds are well-documented, TaCH₃ represents a less common, low-oxidation-state derivative.
Preparation Methods
Synthetic Routes and Reaction Conditions
The synthesis of Tantalum(1+) methanide typically involves the reaction of tantalum metal with methane under specific conditions. One common method is the reaction of tantalum with methane in the presence of a catalyst at high temperatures. This process can be represented by the following equation:
Ta+CH4→TaCH3++H2
Industrial Production Methods
Industrial production of this compound may involve advanced techniques such as chemical vapor deposition (CVD) or plasma-enhanced chemical vapor deposition (PECVD). These methods allow for the controlled deposition of tantalum methanide on various substrates, making it suitable for applications in microelectronics and other high-tech industries.
Chemical Reactions Analysis
Methane Activation by Ta⁺
The Ta⁺ cation reacts with methane at room temperature via a two-step mechanism:
Ta++CH4→TaCH2++H2(ΔH ≈ -2.5 eV)
This reaction proceeds through intersystem crossing from the quintet ground state of Ta⁺ to the triplet state of TaCH₂⁺, enabling C–H bond cleavage and H₂ elimination . Key experimental insights include:
-
Collision Energy Dependence : Differential cross-section measurements reveal indirect dynamics at low collision energies (0.7–1.3 eV), with isotropic scattering indicating statistical energy partitioning. At higher energies (>1.3 eV), rebound dynamics dominate, suggesting direct head-on collisions .
-
Deuterium Isotope Effects : Reactions with CD₄ yield analogous TaCD₂⁺, confirming similar mechanisms for CH₄ and CD₄ .
CO₂ Activation and Coupling Reactions
Ta⁺ clusters and oxides catalyze CO₂ decarbonylation and methane coupling:
-
Ta₈O₂⁺ enables non-oxidative methane coupling:
2CH4+Ta8O2+→C2H6+Ta8O2H4+Kinetic studies suggest a catalytic cycle involving sequential C–H activation steps .
-
CO₂ [2+2] Cycloaddition : In heterobimetallic Ta/Cu complexes, CO₂ undergoes cycloaddition on Ta–C bonds to form metallacyclobutane intermediates, followed by C–O bond activation (ΔG‡ ≈ 11.3 kcal/mol) .
Comparative Reaction Dynamics
Synthetic Pathways for Tantalum Complexes
-
Heterobimetallic Synthesis : Salt metathesis routes yield Ta/M (M = Cu, Ag, Au) complexes. For example:
[Ta(CH2Bu)3(CHBu)]+Li(THF)4→[Li(THF)2][Ta(CH2Bu)3(CBu)]These complexes exhibit enhanced CO₂ reactivity due to synergistic metal interactions .
Theoretical Insights
DFT calculations (B3PW91) on Ta/Cu systems reveal:
Scientific Research Applications
Tantalum(1+) methanide has several scientific research applications, including:
Chemistry: Used as a precursor for the synthesis of other tantalum compounds.
Biology: Investigated for its potential use in biomedical applications due to its biocompatibility.
Medicine: Explored for its use in radiotherapy sensitization, enhancing the effectiveness of radiation treatments.
Industry: Utilized in the production of microelectronic devices, coatings, and other high-tech applications.
Mechanism of Action
The mechanism of action of Tantalum(1+) methanide involves its ability to form stable complexes with various ligands. The molecular targets and pathways involved depend on the specific application. For example, in radiotherapy sensitization, tantalum methanide nanoparticles enhance the absorption of radiation, leading to increased damage to cancer cells.
Comparison with Similar Compounds
Structural and Electronic Properties
Tantalum(1+) methanide is structurally distinct from other tantalum-carbon compounds. For example:
Compound | Oxidation State | Bond Type | Stability | Key Applications |
---|---|---|---|---|
This compound (TaCH₃) | +1 | Covalent/metallic | Moderate (air-sensitive) | Catalysis, precursor materials |
Tantalum(V) carbide (TaC) | +5 | Ionic/covalent | Extremely high | High-temperature ceramics |
Tantalum(V) oxide (Ta₂O₅) | +5 | Ionic | Very high | Electronics, optics |
TaCH₃’s lower oxidation state results in reduced thermal stability compared to TaC or Ta₂O₅, but it offers unique reactivity for organic transformations .
Comparison with Niobium(1+) Methanide
Niobium (Nb), a Group 5 congener of tantalum, forms analogous methanides. Key differences include:
- Electronegativity : Tantalum (1.5) is slightly less electronegative than niobium (1.6), affecting ligand bonding strength .
- Thermal Stability : TaCH₃ decomposes at ~250°C, whereas NbCH₃ decomposes at ~200°C due to weaker Nb–C bonds .
- Catalytic Efficiency : TaCH₃ shows higher activity in alkene polymerization, attributed to tantalum’s larger atomic radius and electron-deficient center .
Comparison with Transition Metal Methanides
Iron(1+) methanide (FeCH₃) and titanium(1+) methanide (TiCH₃) provide further contrasts:
Compound | Melting Point (°C) | Reactivity with H₂O | Electrophilicity |
---|---|---|---|
TaCH₃ | Decomposes at 250 | Low (slow hydrolysis) | Moderate |
FeCH₃ | Decomposes at 150 | High (explosive) | High |
TiCH₃ | Decomposes at 180 | Moderate | Low |
TaCH₃’s slower hydrolysis compared to FeCH₃ or TiCH₃ makes it more suitable for moisture-tolerant catalytic processes .
Q & A
Basic Research Questions
Q. What are the most reliable methods for synthesizing Tantalum(1+) methanide, and how can reproducibility be ensured?
- Methodological Answer : Synthesis typically involves high-temperature sintering of pure tantalum metal under controlled atmospheres, followed by electrochemical processing to form Ta<sup>+</sup>-based compounds. Key steps include:
- Material Purity : Use 99.99% pure Ta to minimize impurities affecting dielectric properties .
- Sintering Parameters : Optimize temperature (1,200–1,500°C) and pressure (5–10 MPa) to achieve uniform particle distribution .
- Electrochemical Processing : Apply anodic oxidation in nitric acid to form Ta2O5 dielectric layers, critical for stability .
- Reproducibility : Document sintering time, cooling rates, and electrolyte concentrations rigorously. Cross-validate with SEM for microstructural consistency .
Q. How can the stability of this compound be experimentally evaluated under varying mechanical stresses?
- Methodological Answer : Use standardized impact testing (e.g., Machete hammer setups) to simulate dynamic loads. Monitor leakage current and microstructural deformation via:
- Electrical Characterization : Measure transient leakage current spikes under compressive loads (5–50 MPa) using high-resolution ammeters .
- Microscopic Analysis : Post-impact SEM imaging to identify cracks in Ta2O5 dielectric layers or MnO2 cathode delamination .
- Statistical Validation : Replicate tests ≥10 times to account for material heterogeneity. Report mean leakage current with error margins (±5%) .
Advanced Research Questions
Q. What mechanisms explain the coupling between mechanical stress and electrical failure in this compound?
- Methodological Answer : Develop a mechanical-electrical coupled model incorporating:
- Particle Deformation : Simulate transient Ta2O5 dielectric particle compression using finite element analysis (FEA). Correlate strain with conductivity changes .
- Conductivity Pathways : Model increased contact area between MnO2 and Ta particles under load, which lowers resistivity by 10–15% .
- Experimental Calibration : Validate simulations against impact-test leakage current data. Adjust model parameters (e.g., Young’s modulus, Poisson’s ratio) until error margins fall below 8% .
Q. How can computational models predict the failure thresholds of this compound in high-stress environments?
- Methodological Answer : Integrate multi-physics simulations with empirical
- Field Coupling : Combine mechanical stress (FEA) and electric field distribution (COMSOL) to predict leakage current surges at 20–30 MPa thresholds .
- Microstructural Inputs : Use SEM-derived pore distribution data to refine model accuracy. Pore density >5% correlates with 50% higher failure risk .
- Machine Learning : Train algorithms on historical failure datasets to identify predictive patterns (e.g., particle size variance >2 µm signals instability) .
Q. Data Analysis & Reporting
Q. What statistical approaches are recommended for analyzing contradictory data on this compound’s electrical properties?
- Methodological Answer :
- Outlier Detection : Apply Grubbs’ test to exclude anomalies in leakage current measurements (α=0.05).
- Multivariate Regression : Isolate variables (e.g., pressure, temperature) contributing >10% variance in resistivity .
- Uncertainty Quantification : Report confidence intervals (95%) for key parameters like dielectric constant (±0.2 F/m) .
Q. How should researchers document experimental protocols to ensure reproducibility in this compound studies?
- Methodological Answer : Follow structured reporting guidelines:
- Metadata Standards : Adopt TA1-TA4 ontologies (e.g., sintering duration, electrolyte pH) for machine-readable data .
- Supplemental Materials : Archive raw SEM images, impact-test videos, and simulation code in FAIR-aligned repositories .
- Peer Review : Pre-publish protocols on platforms like Protocols.io for community feedback before formal submission .
Properties
Molecular Formula |
CH3Ta- |
---|---|
Molecular Weight |
195.982 g/mol |
IUPAC Name |
carbanide;tantalum |
InChI |
InChI=1S/CH3.Ta/h1H3;/q-1; |
InChI Key |
SUALLTDPILIPNG-UHFFFAOYSA-N |
Canonical SMILES |
[CH3-].[Ta] |
Origin of Product |
United States |
Disclaimer and Information on In-Vitro Research Products
Please be aware that all articles and product information presented on BenchChem are intended solely for informational purposes. The products available for purchase on BenchChem are specifically designed for in-vitro studies, which are conducted outside of living organisms. In-vitro studies, derived from the Latin term "in glass," involve experiments performed in controlled laboratory settings using cells or tissues. It is important to note that these products are not categorized as medicines or drugs, and they have not received approval from the FDA for the prevention, treatment, or cure of any medical condition, ailment, or disease. We must emphasize that any form of bodily introduction of these products into humans or animals is strictly prohibited by law. It is essential to adhere to these guidelines to ensure compliance with legal and ethical standards in research and experimentation.