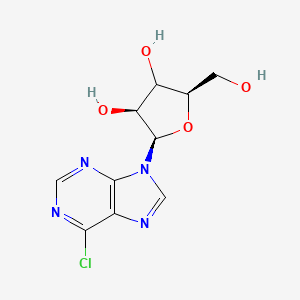
Chloropurine 9-ss-D-ribofuranoside
- Click on QUICK INQUIRY to receive a quote from our team of experts.
- With the quality product at a COMPETITIVE price, you can focus more on your research.
Overview
Description
Chloropurine 9-β-D-ribofuranoside (CAS: 5399-87-1), also referred to as 6-chloropurine-9-β-D-ribofuranoside, is a nucleoside analog featuring a purine base with a chlorine substituent at the 6-position and a β-D-ribofuranosyl sugar moiety attached to the 9-position of the purine ring . This compound is pivotal in medicinal chemistry due to its role as a precursor in synthesizing biologically active nucleosides, such as nebularine (a natural nucleoside with antitumor properties), via catalytic hydrogenation . Its structural flexibility allows for modifications at the 6-position of the purine ring and the sugar moiety, enabling the development of derivatives with varied pharmacological profiles.
Preparation Methods
Synthetic Routes and Reaction Conditions: Chloropurine riboside can be synthesized through various methods. One common approach involves the reaction of chloropurine with ribose or its derivatives under specific conditions. For instance, the reaction can be catalyzed by enzymes such as nucleoside phosphorylases, which facilitate the transglycosylation reaction . Another method involves the use of thermophilic microorganisms like Geobacillus stearothermophilus, which can convert chloropurine to chloropurine riboside with high efficiency .
Industrial Production Methods: Industrial production of chloropurine riboside typically involves large-scale enzymatic synthesis. This method is preferred due to its high yield and specificity. The process involves the use of recombinant enzymes and controlled reaction conditions to ensure the purity and consistency of the product .
Chemical Reactions Analysis
Types of Reactions: Chloropurine riboside undergoes various chemical reactions, including:
Substitution Reactions: The chlorine atom in chloropurine can be substituted with other nucleophiles, such as amines or thiols, under appropriate conditions.
Oxidation and Reduction Reactions: The ribose moiety can undergo oxidation to form ribonolactone derivatives or reduction to form deoxyribose derivatives.
Common Reagents and Conditions:
Substitution Reactions: Common reagents include amines, thiols, and other nucleophiles.
Oxidation and Reduction Reactions: Oxidizing agents like potassium permanganate or reducing agents like sodium borohydride are commonly used.
Major Products:
Substitution Reactions: Products include various substituted purine nucleosides, depending on the nucleophile used.
Oxidation and Reduction Reactions: Products include ribonolactone and deoxyribose derivatives.
Scientific Research Applications
Chemical Properties and Structure
Chloropurine 9-β-D-ribofuranoside is characterized by its unique structure, which includes a chlorinated purine base linked to a ribofuranose sugar. Its chemical formula is C₁₁H₁₄ClN₄O₄. The presence of the chlorine atom at the 6-position of the purine ring significantly influences its biological activity and interaction with various enzymes.
Pharmacological Applications
- Substrate for Enzymatic Reactions :
- Antiviral Activity :
- Cancer Treatment :
Synthetic Chemistry Applications
- Synthesis of Nucleoside Analogues :
- Microwave-Assisted Synthesis :
Biochemical Studies
- Mechanistic Studies :
- Inhibitor Development :
Case Studies and Research Findings
Mechanism of Action
Chloropurine riboside exerts its effects primarily through its interaction with enzymes involved in nucleoside metabolism. It acts as an inhibitor of enzymes such as adenosine deaminase and inosine monophosphate dehydrogenase . By inhibiting these enzymes, chloropurine riboside disrupts the synthesis and metabolism of nucleotides, leading to the inhibition of cell proliferation and induction of apoptosis in cancer cells .
Comparison with Similar Compounds
Comparative Analysis with Structurally Related Compounds
Substituent Variation at the Purine 6-Position
6-Fluoropurine-9-β-D-Ribofuranoside
- Structure : Fluorine replaces chlorine at the 6-position.
- Synthesis : Prepared via direct fluorination of purine derivatives, as demonstrated by Kiburis and Lister (1969), who used hydrogen fluoride or fluorinating agents under controlled conditions .
- Key Differences: The electronegative fluorine atom increases metabolic stability compared to chlorine, reducing susceptibility to hydrolysis. Fluorine’s smaller atomic radius may enhance binding affinity to enzymes like adenosine deaminase.
6-Methylpurine-2′-Deoxy-β-D-Riboside
- Structure: Methyl group at the 6-position; deoxyribose replaces ribofuranose.
- Synthesis : Derived from 6-chloropurine nucleosides via alkylation or enzymatic transglycosylation .
- Deoxyribose reduces steric hindrance, altering interactions with DNA/RNA polymerases .
2,6-Dichloro-9-(β-D-Ribofuranosyl)-9H-Purine
- Structure : Dual chlorine substituents at 2- and 6-positions.
- Synthesis: Synthesized via Vorbrüggen glycosylation of 2,6-dichloropurine with protected ribofuranosyl donors .
- Key Differences: Increased halogen density enhances electrophilicity, making it a potent alkylating agent. Crystal structure analysis reveals an envelope conformation of the ribose ring and absence of π-π stacking interactions, unlike mono-substituted analogs .
Sugar Moiety Modifications
9-(2-Deoxy-2-Fluoro-β-D-Arabinofuranosyl)Purine-6-Thiol
- Structure: Arabinofuranose (C2′-fluoro, C3′-endo conformation) replaces ribofuranose; thiol group at the 6-position.
- Synthesis: Achieved via fluorination of arabinofuranosyl intermediates followed by thiolation .
- Key Differences: The arabinose configuration (2′-fluoro) confers resistance to phosphorylase enzymes, prolonging half-life in vivo. Thiol group enables disulfide bond formation, enhancing targeting of thiol-dependent enzymes .
9-(2,3,5-Tri-O-Acetyl-β-D-Ribofuranosyl)-6-Chloro-9H-Purine
- Structure: Acetyl-protected ribofuranose at 2′,3′,5′-positions.
- Synthesis : Intermediate in nucleoside synthesis; prepared by acetylation of the parent nucleoside .
- Key Differences :
Functionalization at the Purine 2-Position
2-Amino-9-(β-D-Ribofuranosyl) Purine Derivatives
- Structure: Amino group at the 2-position.
- Synthesis : Reacted with aldehydes (e.g., chloroacetaldehyde) to form fluorescent adducts, characterized by NMR and UV spectroscopy .
- Key Differences: The amino group enables hydrogen bonding with nucleic acid bases, mimicking natural nucleosides like adenosine. Fluorescence properties make these derivatives useful as probes in enzymatic assays .
Comparative Data Tables
Table 2: Spectroscopic Data Highlights
Compound | 1H-NMR Shift (Key Protons) | 13C-NMR Shift (Key Carbons) | UV λmax (nm) |
---|---|---|---|
6-Chloropurine-9-β-D-ribofuranoside | δ 8.35 (H8), δ 5.90 (H1′) | δ 152.1 (C6), δ 88.2 (C1′) | 265 |
6-Fluoropurine-9-β-D-ribofuranoside | δ 8.28 (H8), δ 5.88 (H1′) | δ 158.3 (C6), δ 87.9 (C1′) | 260 |
2-Amino-9-β-D-ribofuranosylpurine | δ 7.95 (H8), δ 6.10 (H1′) | δ 156.8 (C2), δ 85.4 (C1′) | 275 |
Biological Activity
Chloropurine 9-β-D-ribofuranoside, a derivative of purine nucleosides, has garnered significant attention in the field of medicinal chemistry and pharmacology due to its diverse biological activities. This article aims to provide a comprehensive overview of its biological properties, synthesis, and potential applications based on recent research findings.
Chloropurine 9-β-D-ribofuranoside is characterized by the presence of a chlorinated purine base linked to a ribofuranose sugar. The synthesis of this compound typically involves the glycosylation of 6-chloropurine with ribofuranose, which can be achieved through various methods including enzymatic and chemical approaches. A notable synthetic route has been reported that enhances yield and efficiency by utilizing selective glycosylation techniques .
Biological Activity
1. Antitumor Activity
Chloropurine derivatives have been evaluated for their antitumor properties. Research indicates that chloropurine 9-β-D-ribofuranoside exhibits cytotoxic effects against various cancer cell lines. In vitro studies have shown that this compound can inhibit cell proliferation and induce apoptosis in human leukemia cells more effectively than some parent compounds . The mechanism of action is believed to involve the disruption of nucleic acid synthesis, leading to cell cycle arrest.
2. Cytokinin Activity
As a cytokinin analogue, chloropurine 9-β-D-ribofuranoside has been investigated for its role in plant growth regulation. It has been found to promote cell division and differentiation in plant tissues, thereby enhancing growth and development under controlled conditions. This activity is attributed to its interaction with cytokinin receptors, which are pivotal in mediating plant responses to environmental stimuli .
3. Immunomodulatory Effects
In addition to its antitumor and cytokinin activities, chloropurine 9-β-D-ribofuranoside has shown potential as an immunomodulatory agent. Studies suggest that it may enhance immune responses by modulating cytokine production in immune cells, thereby offering therapeutic avenues for conditions requiring immune system support .
Case Studies
Several case studies have highlighted the efficacy of chloropurine derivatives in clinical settings:
- Leukemia Treatment : A study involving patients with acute lymphoblastic leukemia demonstrated that chloropurine derivatives could be integrated into treatment regimens, resulting in improved patient outcomes due to their enhanced cytotoxicity against malignant cells .
- Plant Growth Trials : Field trials conducted on crops treated with chloropurine 9-β-D-ribofuranoside showed significant increases in yield and resistance to stress conditions, indicating its practical applications in agriculture as a growth promoter .
Summary of Research Findings
Q & A
Basic Research Questions
Q. What are the established synthetic routes for Chloropurine 9-ss-D-ribofuranoside, and how do reaction conditions influence yield and purity?
this compound is synthesized via nucleoside coupling reactions, often involving purine bases and protected ribofuranosyl derivatives. Key steps include:
- Halogenation : Introduction of chlorine at the purine C6 position using agents like POCl₃ under controlled temperatures (0–5°C) to minimize side reactions .
- Glycosylation : Coupling the chlorinated purine with a protected ribofuranosyl donor (e.g., 1-O-acetyl-2,3,5-tri-O-benzoyl-β-D-ribofuranose) in the presence of Lewis acids (e.g., TMSOTf) to form the β-D-ribofuranoside linkage. Yield optimization requires anhydrous conditions and inert atmospheres .
- Deprotection : Sequential removal of protecting groups (e.g., benzoyl or acetyl groups) using alkaline hydrolysis (e.g., NH₃/MeOH) to yield the final compound. Purity is monitored via HPLC (C18 column, UV detection at 254 nm) .
Q. Which analytical techniques are most reliable for characterizing this compound, and how are spectral data interpreted?
- NMR Spectroscopy : ¹H and ¹³C NMR confirm the ribofuranosyl anomeric configuration (β-D configuration: J₁',2' ≈ 4–6 Hz) and chlorine substitution at C6 (downfield shift of purine protons) .
- Mass Spectrometry : High-resolution MS (HRMS-ESI) validates molecular weight (e.g., [M+H]⁺ at m/z 301.0452 for C₁₀H₁₂ClN₄O₄) .
- HPLC-PDA : Reverse-phase chromatography with photodiode array detection assesses purity (>98%) and identifies degradation products under stress conditions (e.g., acidic/basic hydrolysis) .
Q. How does the compound’s stability vary under different pH and temperature conditions, and what storage protocols are recommended?
Stability studies show:
- pH Sensitivity : Degrades rapidly in alkaline conditions (pH > 9) via glycosidic bond hydrolysis. Acidic conditions (pH < 3) induce purine ring protonation, reducing solubility .
- Thermal Stability : Stable at 4°C for 6 months in lyophilized form. Aqueous solutions degrade within 72 hours at 25°C, necessitating −20°C storage in amber vials .
Advanced Research Questions
Q. What mechanistic insights exist regarding this compound’s antiviral activity, and how can conflicting data from in vitro vs. in vivo models be resolved?
The compound inhibits viral polymerases by mimicking natural nucleosides, causing chain termination. Contradictions arise due to:
- Metabolic Differences : In vivo hepatic conversion to inactive metabolites (e.g., 6-chloropurine) reduces efficacy compared to in vitro systems .
- Experimental Design : Use embedded models (e.g., combining quantitative polymerase inhibition assays with qualitative metabolite profiling) to reconcile discrepancies .
Q. How do structural modifications (e.g., C2/C6 functionalization) impact this compound’s bioactivity, and what computational tools guide rational design?
- C2 Vinylation : Enhances lipophilicity, improving blood-brain barrier penetration but reducing solubility. Molecular docking (AutoDock Vina) predicts binding affinity shifts in viral targets .
- C6 Thiolation : Increases metabolic stability but may introduce toxicity. Density Functional Theory (DFT) calculations optimize electronic properties for target engagement .
Q. What methodologies are recommended for studying the compound’s interaction with human enzymes (e.g., adenosine deaminase) to assess therapeutic potential?
- Kinetic Assays : Measure IC₅₀ values via spectrophotometric monitoring of adenosine deaminase activity (UV absorbance at 265 nm) .
- Isothermal Titration Calorimetry (ITC) : Quantifies binding thermodynamics (ΔH, ΔS) to identify competitive vs. non-competitive inhibition .
Q. How should researchers address contradictions in cytotoxicity data across cell lines, and what controls are essential?
- Cell-Specific Factors : Variations in nucleoside transporter expression (e.g., hENT1) affect intracellular uptake. Validate using transporter inhibitors (e.g., dipyridamole) .
- Standardized Controls : Include 5-fluorouracil as a positive control and untreated cells for baseline apoptosis rates (flow cytometry with Annexin V/PI staining) .
Q. Methodological Frameworks
- Experimental Design : Use a hybrid embedded design (quantitative primary + qualitative secondary questions) to address multi-layered hypotheses .
- Data Interpretation : Apply the FINER criteria (Feasible, Interesting, Novel, Ethical, Relevant) to evaluate research questions and resolve contradictions .
- Theoretical Alignment : Link studies to nucleoside analog theory or viral replication mechanisms to contextualize findings .
Properties
Molecular Formula |
C10H11ClN4O4 |
---|---|
Molecular Weight |
286.67 g/mol |
IUPAC Name |
(2R,3S,5R)-2-(6-chloropurin-9-yl)-5-(hydroxymethyl)oxolane-3,4-diol |
InChI |
InChI=1S/C10H11ClN4O4/c11-8-5-9(13-2-12-8)15(3-14-5)10-7(18)6(17)4(1-16)19-10/h2-4,6-7,10,16-18H,1H2/t4-,6?,7+,10-/m1/s1 |
InChI Key |
XHRJGHCQQPETRH-HMEJCUHCSA-N |
Isomeric SMILES |
C1=NC2=C(C(=N1)Cl)N=CN2[C@H]3[C@H](C([C@H](O3)CO)O)O |
Canonical SMILES |
C1=NC2=C(C(=N1)Cl)N=CN2C3C(C(C(O3)CO)O)O |
Origin of Product |
United States |
Disclaimer and Information on In-Vitro Research Products
Please be aware that all articles and product information presented on BenchChem are intended solely for informational purposes. The products available for purchase on BenchChem are specifically designed for in-vitro studies, which are conducted outside of living organisms. In-vitro studies, derived from the Latin term "in glass," involve experiments performed in controlled laboratory settings using cells or tissues. It is important to note that these products are not categorized as medicines or drugs, and they have not received approval from the FDA for the prevention, treatment, or cure of any medical condition, ailment, or disease. We must emphasize that any form of bodily introduction of these products into humans or animals is strictly prohibited by law. It is essential to adhere to these guidelines to ensure compliance with legal and ethical standards in research and experimentation.