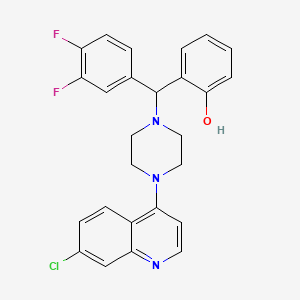
Cysteine protease inhibitor-3
- Click on QUICK INQUIRY to receive a quote from our team of experts.
- With the quality product at a COMPETITIVE price, you can focus more on your research.
Overview
Description
Cysteine protease inhibitor-3 is a synthetic small-molecule inhibitor with the molecular formula C₂₆H₂₂ClF₂N₃O and a molecular weight of 465.92 g/mol . It is widely used in research to modulate cysteine protease activity, particularly in studies related to cell death mechanisms, immunology, and neuroendocrine signaling . The compound is stable in powder form at -20°C for up to 3 years and requires reconstitution in solvents like DMSO for experimental use . While its exact biological targets remain unspecified in available literature, its structural features (e.g., a nitrile group) suggest a mechanism involving covalent binding to the catalytic cysteine residue of proteases, a common strategy among cysteine protease inhibitors .
Preparation Methods
Synthetic Routes and Reaction Conditions
The synthesis of cysteine protease inhibitor-3 typically involves the use of peptide synthesis techniques. The process begins with the selection of appropriate amino acid residues, which are then sequentially coupled using solid-phase peptide synthesis. The reaction conditions often include the use of coupling reagents such as dicyclohexylcarbodiimide and N-hydroxysuccinimide to facilitate peptide bond formation. The final product is purified using high-performance liquid chromatography.
Industrial Production Methods
Industrial production of this compound involves large-scale peptide synthesis using automated synthesizers. The process is optimized for high yield and purity, with stringent quality control measures in place to ensure consistency. The final product is typically lyophilized and stored under controlled conditions to maintain stability.
Chemical Reactions Analysis
Types of Reactions
Cysteine protease inhibitor-3 undergoes various chemical reactions, including:
Oxidation: The thiol group of the cysteine residue can be oxidized to form disulfide bonds.
Reduction: Disulfide bonds can be reduced back to thiol groups using reducing agents such as dithiothreitol.
Substitution: The inhibitor can undergo nucleophilic substitution reactions, particularly at the peptide bonds.
Common Reagents and Conditions
Oxidation: Hydrogen peroxide or other oxidizing agents.
Reduction: Dithiothreitol or β-mercaptoethanol.
Substitution: Nucleophiles such as amines or thiols under mild conditions.
Major Products
The major products formed from these reactions include oxidized or reduced forms of the inhibitor, as well as substituted derivatives with modified peptide bonds.
Scientific Research Applications
Cancer Therapy
CPI-3 has shown promise in cancer treatment, particularly in inhibiting tumor metastasis.
- Mechanism of Action : CPI-3 selectively inhibits cathepsin L, a cysteine protease associated with tumor invasion and metastasis. Studies indicate that CPI-3 can reduce the migration and invasion of breast cancer cells (MDA-MB-231), demonstrating its potential as an anti-cancer agent .
- Case Study : A study characterized the inhibitory activity of SnuCalCpI15, a variant of CPI-3, revealing an inhibitory constant (Ki) of 1.38 nM against cathepsin L. This suggests a strong potential for CPI-3 in preventing breast cancer metastasis .
Cysteine Protease | Inhibitory Constant (nM) | Effect on Cancer Cells |
---|---|---|
Cathepsin L | 1.38 | Reduced migration/invasion |
Cathepsin B | 2.50 | Moderate effect |
Infectious Diseases
CPI-3 has applications in combating infectious diseases caused by parasites and viruses.
- Parasitic Infections : Research has highlighted the role of cysteine proteases in Trypanosoma brucei and Trypanosoma cruzi, which are responsible for diseases like sleeping sickness and Chagas disease. CPI-3's inhibition of these proteases may disrupt the parasite's lifecycle, offering a novel therapeutic strategy .
- Case Study : A covalent inhibitor targeting cysteine proteases was tested in preclinical trials, showing safety across various mammalian models while effectively reducing parasite viability .
Neurodegenerative Disorders
CPI-3 is being explored for its potential in treating neurodegenerative diseases such as Huntington's disease.
- Mechanism of Action : Inhibition of caspases (cysteine proteases involved in apoptosis) can protect neurons from degeneration. Novel pan-caspase inhibitors derived from CPI-3 have shown efficacy in reducing toxicity associated with Huntington's disease models .
Disease | Target Enzyme | Effectiveness |
---|---|---|
Huntington's Disease | Caspases | Reduced neurotoxicity |
Therapeutic Development
The development of CPI-3 derivatives is ongoing, focusing on enhancing their specificity and potency.
Mechanism of Action
Cysteine protease inhibitor-3 exerts its effects by binding to the active site of cysteine proteases, thereby blocking their catalytic activity. The inhibitor forms a covalent bond with the thiol group of the cysteine residue in the enzyme’s active site, preventing substrate binding and subsequent proteolysis. This interaction is highly specific and reversible, allowing for precise regulation of protease activity.
Comparison with Similar Compounds
Below is a detailed comparison with key analogues:
Table 1: Comparative Analysis of Cysteine Protease Inhibitor-3 and Related Compounds
Key Insights:
Mechanistic Diversity :
- Covalent vs. Competitive Inhibition : While this compound and nitrile-based inhibitors (e.g., E-64 derivatives) act via covalent modification of catalytic cysteines , RMS-3 inhibits serine proteases through substrate competition .
- Electrophilic Warheads : Falcipain inhibitors employ soft electrophiles (e.g., vinyl sulfones) for irreversible inhibition, minimizing off-target effects in antimalarial applications .
Human vs. Pathogen Targets: Falcipain inhibitors exhibit selectivity for Plasmodium proteases over human homologs (e.g., cathepsin K), a critical feature for antimalarial safety .
Therapeutic Applications: Disease-Specific Design: Caspase-6 inhibitor 3a leverages a non-catalytic cysteine residue for selective modulation, offering promise in Alzheimer’s disease . Broad-Spectrum Utility: this compound’s inferred broad-spectrum activity makes it a versatile tool for exploratory research, though its lack of target specificity limits therapeutic use .
Structural and Computational Advances: Nitrile-Based Inhibitors: Molecular dynamics simulations reveal that nitrile groups form stable thioimidate adducts with catalytic cysteines, enhancing inhibitory potency . Falcipain Inhibitors: Structure-based virtual screening identified nonpeptidic scaffolds with improved metabolic stability, achieving a 42% hit rate in FP-2 inhibition .
Biological Activity
Cysteine protease inhibitor-3 (CPI-3), also known as cystatin C, is a crucial member of the cystatin family of proteins that inhibit cysteine proteases. This article explores the biological activity of CPI-3, focusing on its mechanisms of action, therapeutic potential, and implications in various diseases.
Overview of this compound
CPI-3 is primarily involved in regulating proteolytic processes by inhibiting cysteine proteases such as cathepsins. These enzymes play significant roles in various biological functions, including protein degradation, cell signaling, and apoptosis. The inhibition of cysteine proteases by CPI-3 is essential for maintaining cellular homeostasis and has been implicated in several pathological conditions.
CPI-3 inhibits cysteine proteases through competitive binding to the active site of these enzymes. The inhibition mechanism can be influenced by factors such as pH and the presence of other proteins. Research indicates that CPI-3 forms stable complexes with target proteases, thereby preventing substrate access and subsequent proteolytic activity.
Key Findings:
- Binding Affinity : CPI-3 exhibits high binding affinity for cathepsin L and other cysteine proteases, which is crucial for its inhibitory function .
- Dimerization : The formation of disulfide-bonded dimers enhances the inhibitory potency of CPI-3 against certain proteases, suggesting that conformational changes may impact its activity .
Biological Activities and Therapeutic Implications
CPI-3 has been studied for its role in various biological processes and diseases:
- Cancer : CPI-3 has shown potential as an anti-cancer agent by inhibiting tumor cell invasion and migration. Studies indicate that CPI-3 can reduce the activity of cathepsin L in breast cancer cells, leading to decreased metastatic potential .
- Inflammation : By modulating the activity of cysteine proteases involved in inflammatory responses, CPI-3 may play a protective role in inflammatory diseases. For instance, tick-derived cysteine protease inhibitors have demonstrated immunomodulatory effects that can suppress inflammation .
- Neuroprotection : There is emerging evidence that CPI-3 may protect against neurodegenerative diseases by inhibiting proteolytic enzymes that contribute to neuronal damage .
Case Study 1: CPI-3 in Breast Cancer
A study evaluated the effects of CPI-3 on MDA-MB-231 breast cancer cells. Treatment with CPI-3 resulted in over a 20% reduction in cathepsin L activity within both intracellular and extracellular environments, indicating its potential to inhibit tumor progression effectively .
Case Study 2: Immunomodulatory Effects
Research on tick cystatin inhibitors revealed their ability to modulate immune responses during psoriasis-like inflammation. Mice treated with these inhibitors showed reduced skin thickness and inflammation markers compared to control groups .
Data Summary
Q & A
Q. How can researchers determine the in vitro efficacy of Cysteine protease inhibitor-3 against Plasmodium falciparum strains?
Answer:
To assess efficacy, measure the half-maximal inhibitory concentration (IC50) using standardized enzyme activity assays. Prepare serial dilutions of the inhibitor and incubate with target proteases (e.g., PfFP2, PfFP3) or cultured parasites. Quantify residual protease activity via fluorometric or colorimetric substrates. For Plasmodium strains (e.g., Pf3D7, PfW2), IC50 values can be determined using dose-response curves. For example, this compound exhibits IC50 values of 0.74 µM (Pf3D7) and 1.05 µM (PfW2) . Use statistical tools (e.g., GraphPad Prism) to calculate mean ± SD and perform significance testing (e.g., ANOVA) to validate reproducibility .
Q. What computational strategies are employed to optimize the binding affinity and selectivity of this compound?
Answer:
Structure-based drug design (SBDD) and molecular dynamics (MD) simulations are critical. Utilize X-ray crystallography or cryo-EM structures of target proteases to identify binding pockets. Software like AutoDock or Schrödinger Suite can predict inhibitor-enzyme interactions. For covalent inhibitors, simulate nucleophilic attack mechanisms at the catalytic cysteine residue. Advanced MD simulations (e.g., Gaussian accelerated MD) can assess binding stability and residence time. These methods were pivotal in optimizing dipeptide inhibitors with enhanced metabolic stability and cell permeability .
Q. How should researchers resolve contradictions between in vitro specificity and observed cross-reactivity in cellular assays?
Answer:
Cross-reactivity often arises from off-target interactions with structurally similar proteases (e.g., caspases or cathepsins). Validate specificity using orthogonal assays:
- Biochemical assays: Test inhibitor activity against a panel of recombinant proteases.
- Cellular assays: Combine inhibitor treatment with RNAi/CRISPR knockdown of target proteases to confirm on-target effects.
- Proteome-wide profiling: Use chemical proteomics (e.g., activity-based protein profiling) to identify off-target interactions .
For example, pan-caspase inhibitors like Z-VAD-FMK show cross-reactivity with caspases-3/7/8/9, necessitating complementary genetic validation .
Q. What methodological considerations are critical when translating in vitro efficacy of this compound to in vivo models?
Answer:
Key steps include:
- Formulation optimization: Use biocompatible solvents (e.g., 5% DMSO + 30% PEG300 + 5% Tween 80) to enhance solubility and bioavailability .
- Dose calibration: Adjust doses based on animal weight (e.g., 10 mg/kg for a 20 g mouse) and pharmacokinetic (PK) parameters (e.g., half-life, clearance).
- Toxicity screening: Monitor for off-target effects in vital organs (e.g., liver, kidneys) using histopathology and serum biomarkers.
- PK/PD modeling: Corrogate plasma concentration-time profiles with target engagement using LC-MS/MS or fluorescence imaging .
Q. How can researchers validate the purity and molecular weight of this compound post-synthesis?
Answer:
- Analytical HPLC: Use a C18 column with UV detection (λ = 254 nm) to assess purity (>95%).
- Mass spectrometry (MS): Confirm molecular weight (465.92 Da for C26H22ClF2N3O) via ESI-TOF or MALDI-TOF .
- SDS-PAGE: For protein-based inhibitors, resolve samples on 12–15% gels and stain with Coomassie Blue to verify a single band (e.g., 20 kDa for a purified inhibitor) .
Q. What statistical approaches are essential for analyzing enzyme inhibition data?
Answer:
- Dose-response curves: Fit data to a four-parameter logistic model (e.g., Hill equation) to derive IC50 values.
- Error analysis: Calculate standard deviation (SD) across triplicates and perform outlier tests (e.g., Grubbs’ test).
- Significance testing: Use Student’s t-test or one-way ANOVA (with Tukey’s post-hoc) to compare treatment groups .
Q. How can researchers address metabolic instability of this compound in in vivo studies?
Answer:
- Structural modification: Introduce metabolically stable groups (e.g., fluorinated aryl rings, methylphosphonate esters) to reduce CYP450-mediated degradation .
- Prodrug design: Mask reactive groups (e.g., ketones) with enzymatically cleavable moieties (e.g., ester prodrugs).
- Co-administration: Combine with cytochrome P450 inhibitors (e.g., ketoconazole) to prolong half-life .
Q. What advanced techniques are used to study the covalent binding mechanism of this compound?
Answer:
- X-ray crystallography: Resolve inhibitor-enzyme complexes to identify covalent adducts (e.g., thioether bonds).
- Isothermal titration calorimetry (ITC): Measure binding thermodynamics (ΔG, ΔH) to assess covalent vs. non-covalent interactions.
- Quantum mechanics/molecular mechanics (QM/MM): Model electron transfer during covalent bond formation .
Q. How should researchers design experiments to evaluate resistance mechanisms against this compound?
Answer:
- Serial passage assays: Culture parasites or cells under sublethal inhibitor pressure for 10–20 generations.
- Whole-genome sequencing: Identify mutations in target proteases (e.g., PfFP2 S148L) or efflux pumps (e.g., PfMDR1).
- Competitive fitness assays: Compare growth rates of resistant vs. wild-type strains in co-cultures .
Q. What are the best practices for long-term storage of this compound?
Answer:
Properties
Molecular Formula |
C26H22ClF2N3O |
---|---|
Molecular Weight |
465.9 g/mol |
IUPAC Name |
2-[[4-(7-chloroquinolin-4-yl)piperazin-1-yl]-(3,4-difluorophenyl)methyl]phenol |
InChI |
InChI=1S/C26H22ClF2N3O/c27-18-6-7-19-23(16-18)30-10-9-24(19)31-11-13-32(14-12-31)26(20-3-1-2-4-25(20)33)17-5-8-21(28)22(29)15-17/h1-10,15-16,26,33H,11-14H2 |
InChI Key |
VBYITYHKLOIIBW-UHFFFAOYSA-N |
Canonical SMILES |
C1CN(CCN1C2=C3C=CC(=CC3=NC=C2)Cl)C(C4=CC(=C(C=C4)F)F)C5=CC=CC=C5O |
Origin of Product |
United States |
Disclaimer and Information on In-Vitro Research Products
Please be aware that all articles and product information presented on BenchChem are intended solely for informational purposes. The products available for purchase on BenchChem are specifically designed for in-vitro studies, which are conducted outside of living organisms. In-vitro studies, derived from the Latin term "in glass," involve experiments performed in controlled laboratory settings using cells or tissues. It is important to note that these products are not categorized as medicines or drugs, and they have not received approval from the FDA for the prevention, treatment, or cure of any medical condition, ailment, or disease. We must emphasize that any form of bodily introduction of these products into humans or animals is strictly prohibited by law. It is essential to adhere to these guidelines to ensure compliance with legal and ethical standards in research and experimentation.