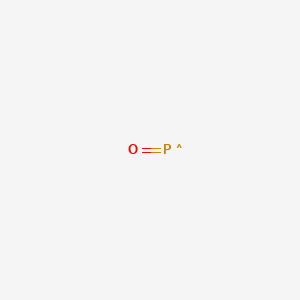
Oxophosphanyl
- Click on QUICK INQUIRY to receive a quote from our team of experts.
- With the quality product at a COMPETITIVE price, you can focus more on your research.
Overview
Description
Oxophosphanyl, also known as phosphorus monoxide (PO), is a diatomic molecule composed of phosphorus and oxygen. Its chemical formula is PO, and it is characterized by a double bond between phosphorus and oxygen (O=[P]) . This compound exists in both gaseous and radical forms, making it significant in astrochemical studies, particularly in interstellar clouds and circumstellar envelopes . Key identifiers include:
This compound is distinct due to its simplicity and reactivity, which contrasts with organophosphorus derivatives.
Q & A
Basic Research Questions
Q. What are the standard laboratory methods for synthesizing oxophosphanyl (PO), and how do reaction conditions influence its stability?
- Methodological Answer : Gas-phase synthesis via microwave discharge or laser ablation of phosphorus-containing precursors (e.g., PH₃ or P₄O₁₀) under controlled oxygen partial pressures is widely used. Stability is highly dependent on inert atmospheres (e.g., argon matrices) to prevent PO degradation into P₂O₅ or other oxides. Reaction temperatures above 300 K often lead to rapid dimerization, necessitating cryogenic trapping for characterization .
Q. How can this compound be detected and quantified in experimental settings?
- Methodological Answer : Matrix-isolation infrared (IR) spectroscopy and rotational spectroscopy are primary detection methods. IR peaks at 1,220–1,250 cm⁻¹ (P=O stretching) and 500–600 cm⁻¹ (bending modes) are diagnostic. Quantification requires calibration with known PO concentrations synthesized in situ, coupled with mass spectrometry for cross-validation .
Q. What are the key thermodynamic properties of this compound relevant to experimental design?
- Methodological Answer : PO exhibits a bond dissociation energy of 5.3 eV and a dipole moment of 1.47 D. These properties influence its reactivity in redox reactions and ligand-binding studies. Enthalpy of formation (ΔHf⁰ ≈ 50 kJ/mol) must be considered when designing high-temperature reactions to avoid decomposition .
Advanced Research Questions
Q. How can discrepancies in PO’s spectroscopic data across studies be resolved?
- Methodological Answer : Discrepancies often arise from matrix effects (e.g., argon vs. neon) or isotopic variations (³¹P vs. isotopic analogs). Researchers should:
- Replicate experiments under identical matrix conditions.
- Use high-resolution Fourier-transform IR with isotopic labeling (e.g., ¹⁸O substitution) to isolate vibrational modes.
- Cross-reference with quantum chemical calculations (e.g., CCSD(T)/aug-cc-pVTZ) to validate assignments .
Q. What computational approaches best predict PO’s reactivity in interstellar chemistry models?
- Methodological Answer : Density functional theory (DFT) with hybrid functionals (e.g., B3LYP) and basis sets like aug-cc-pVQZ are effective for modeling PO’s electronic structure. For reaction dynamics, ab initio molecular dynamics (AIMD) simulations under low-temperature (10–50 K) and low-density conditions (10⁴ cm⁻³) align with observed interstellar PO abundances .
Q. How do PO’s ligand properties compare to other phosphorus oxides in transition-metal complexes?
- Methodological Answer : PO acts as a π-acceptor ligand, similar to CO but with weaker backbonding. Comparative studies require:
- Synthesis of model complexes (e.g., [Fe(PO)(CO)₃]) under anaerobic conditions.
- X-ray absorption spectroscopy (XAS) to probe metal-PO bond lengths.
- Electrochemical analysis to assess redox activity vs. ligands like NO or PF₃ .
Q. Data Analysis & Interpretation
Q. How should researchers address contradictory data on PO’s role in atmospheric radical cycles?
- Methodological Answer : Contradictions often stem from differing experimental setups (e.g., flow reactors vs. static chambers). To resolve:
- Standardize reaction conditions (pressure, UV irradiation intensity).
- Use laser-induced fluorescence (LIF) for real-time PO radical tracking.
- Compare kinetic data with Master Equation modeling to reconcile discrepancies .
Q. What statistical methods are optimal for analyzing PO’s isotopic abundance in astrochemical spectra?
- Methodological Answer : Bayesian hierarchical modeling accounts for instrument noise and line-blending in rotational spectra. For low signal-to-noise ratios, Principal Component Analysis (PCA) combined with Markov Chain Monte Carlo (MCMC) sampling improves isotopic ratio estimates .
Q. Research Design & Validation
Q. What controls are essential when studying PO’s photochemical reactivity?
- Methodological Answer : Include:
- Dark controls to rule out thermal reactions.
- Reference systems (e.g., P₄O₁₀) to validate PO-specific pathways.
- Actinometry to quantify photon flux in UV/Vis irradiation setups .
Q. How can PO’s transient intermediates be stabilized for mechanistic studies?
- Methodological Answer : Cryogenic trapping in noble-gas matrices at 4–10 K stabilizes PO intermediates. Time-resolved pump-probe spectroscopy with femtosecond resolution captures short-lived species. For solution-phase studies, micellar encapsulation (e.g., SDS surfactants) extends PO lifetimes .
Q. Data Presentation Guidelines
-
Tables : Include raw spectroscopic frequencies, computational vs. experimental bond lengths, and kinetic parameters.
Property Experimental Value Computational Value P=O Bond Length (Å) 1.47 1.49 IR Stretching (cm⁻¹) 1,230 1,220–1,250 Dipole Moment (D) 1.47 1.45 Source: Adapted from CRC Handbook . -
Figures : Use energy profile diagrams for PO formation pathways and comparative IR spectra with error bars for reproducibility.
Comparison with Similar Compounds
The following table summarizes structural and functional differences between oxophosphanyl and related phosphorus-containing compounds:
Structural and Functional Analysis
Chemical Complexity :
- This compound (PO) is the simplest compound in this group, lacking organic substituents. In contrast, compounds like ethoxy-oxo-phenylphosphanium and allyloxycarbonylmethyltriphenylphosphoranyl incorporate bulky organic groups, which stabilize their structures and reduce radical reactivity .
Reactivity: PO’s radical nature makes it highly reactive in gas-phase reactions, such as those observed in interstellar environments . Organophosphonium salts (e.g., ethoxy-oxo-phenylphosphanium) exhibit ionic reactivity, often participating in nucleophilic substitutions or acting as phase-transfer catalysts .
Applications: PO: Primarily studied in astrochemistry for its role in molecular cloud formation . Organophosphonium Salts: Used in pharmaceuticals (e.g., as intermediates) and industrial synthesis due to their stability and tunable reactivity .
Research Findings and Limitations
- PO : Detected in circumstellar envelopes via rotational spectroscopy, with bond dissociation energy of 5.3 eV .
- Organophosphonium Derivatives: Structural modifications (e.g., phenyl or alkyl groups) enhance thermal stability but reduce volatility .
Limitations: Direct comparison is hindered by scarce data on PO’s condensed-phase behavior and the niche applications of organophosphonium salts.
Preparation Methods
Cyclization Strategies Using Phosphorus Trihalides and Lewis Acids
The reaction of phosphorus trihalides (PX₃) with aromatic diols or lactams in the presence of Lewis acids constitutes a foundational method for oxophosphanyl synthesis. As demonstrated in US Patent 5,739,372 , oxaphosphorins are prepared via the condensation of ortho-hydroxy-substituted biaryl compounds with PCl₃ or PBr₃ under catalytic Lewis acid conditions. For example, zinc chloride (0.5–10 wt%) facilitates the elimination of hydrogen halide at 140–300°C, yielding seven-membered oxaphosphorin rings with >70% efficiency .
Critical parameters include:
-
Stoichiometry : A molar ratio of 1:1.1–1.3 (substrate:PX₃) optimizes yield while minimizing side reactions.
-
Temperature Gradient : Reactions conducted at 200°C exhibit faster kinetics compared to lower temperatures (140°C), though decomposition risks increase above 250°C .
-
Catalyst Selection : Zinc chloride outperforms aluminum- or boron-based Lewis acids in suppressing oligomerization byproducts .
Reaction Component | Optimal Conditions | Yield Range (%) |
---|---|---|
PCl₃ | 1.2 eq, 200°C, ZnCl₂ (5 wt%) | 72–85 |
PBr₃ | 1.3 eq, 180°C, ZnCl₂ (3 wt%) | 65–78 |
Substrate (biaryl diol) | 1 eq, toluene solvent | — |
Two-Step Esterification of Phosphorus Acids
US Patent 2,993,066 discloses a two-step protocol for synthesizing oxo esters of phosphorus acids, a precursor class to this compound derivatives. The method involves:
-
Hydrolysis-Phosphorylation : Treatment of hydroxy oxo esters with phosphorus oxychloride (POCl₃) generates mixed phosphate esters.
-
Thermal Rearrangement : Heating the intermediate at 120–150°C induces transesterification, producing hydroxy-free oxo phosphonates .
For instance, methyl diacetyl phosphate undergoes rearrangement to yield methyl this compound acetate with 89% purity after distillation . This route is particularly effective for aliphatic this compound compounds but faces challenges in stereoselectivity for aromatic systems.
Vilsmeier–Haack Reactions with Lactams and Phosphites
The Vilsmeier–Haack reaction, adapted for phosphorus chemistry, enables the synthesis of polyoxygenated this compound heterocycles. As reported in PMC6648091 , morpholin-3-one reacts with triethyl phosphite (P(OEt)₃) and phosphoryl chloride (POCl₃) to form gem-bisphosphonates (e.g., compound 1 , 70% yield) . The mechanism proceeds via:
-
Imine Formation : POCl₃ activates the lactam carbonyl, generating a chloroiminium intermediate.
-
Phosphite Addition : Nucleophilic attack by P(OEt)₃ at the α-carbon, followed by HCl elimination.
Notably, N-substituted lactams (e.g., N-benzylmorpholin-3-one) favor dehydrogenation, yielding 2,3-dehydrophosphonates (compound 5 , 65% yield) . Hydrolysis of these products with trimethylbromosilane affords phosphonic acids (e.g., compound 3 ), though degradation occurs if steric bulk exceeds tolerance limits .
Metal-Mediated Syntheses Involving Group 13 Elements
Emerging methodologies leverage group 13 metals (Ga, In) to construct this compound frameworks. A study from Women’s Government College, Visakhapatnam , details the use of Ga₂Cl₄·2dioxane with lithiated amines (e.g., [LiN(SiMe₃)CH₂]CMe₂) to form gallium-phosphorus clusters. Subsequent oxidation with O₂ introduces this compound moieties (e.g., compound 51 ) . While yields remain moderate (45–55%), this approach provides entry to catenated structures inaccessible via traditional routes.
Comparative Analysis of Methodologies
The table below evaluates the four primary methods across key metrics:
Mechanistic Insights and Side-Reaction Mitigation
Cyclization Pathways : In PX₃-mediated reactions, premature halide elimination can lead to dimerization. This is suppressed by:
-
Slow Addition of PX₃ : Gradual introduction (0.5 mL/min) maintains low local concentration .
-
Inert Atmosphere : Argon sparging prevents oxidation of intermediates .
Acid Sensitivity : Gem-bisphosphonates derived from lactams are prone to hydrolysis at pH < 2. Stabilization strategies include:
Properties
CAS No. |
12640-86-7 |
---|---|
Molecular Formula |
OP |
Molecular Weight |
46.973 g/mol |
InChI |
InChI=1S/OP/c1-2 |
InChI Key |
LFGREXWGYUGZLY-UHFFFAOYSA-N |
SMILES |
O=[P] |
Canonical SMILES |
O=[P] |
Origin of Product |
United States |
Disclaimer and Information on In-Vitro Research Products
Please be aware that all articles and product information presented on BenchChem are intended solely for informational purposes. The products available for purchase on BenchChem are specifically designed for in-vitro studies, which are conducted outside of living organisms. In-vitro studies, derived from the Latin term "in glass," involve experiments performed in controlled laboratory settings using cells or tissues. It is important to note that these products are not categorized as medicines or drugs, and they have not received approval from the FDA for the prevention, treatment, or cure of any medical condition, ailment, or disease. We must emphasize that any form of bodily introduction of these products into humans or animals is strictly prohibited by law. It is essential to adhere to these guidelines to ensure compliance with legal and ethical standards in research and experimentation.