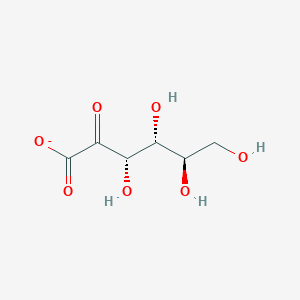
2-dehydro-D-gluconate
Overview
Description
2-Dehydro-D-gluconate (C₆H₁₀O₇), also known as 2-keto-D-gluconic acid, is a sugar acid derivative involved in microbial metabolism and eukaryotic energy pathways. It is a key intermediate in the pentose phosphate pathway (PPP) and the Entner-Doudoroff (ED) pathway, where it participates in redox reactions and energy production . Structurally, it is characterized by a keto group at the C2 position of the gluconate backbone, distinguishing it from other sugar acids like D-gluconate and 5-dehydro-D-gluconate . Recent studies highlight its role in diverse biological contexts, including fertility regulation in cattle , microbial decomposition , and industrial biotechnology .
Scientific Research Applications
Enzymatic Reactions
The enzymatic conversion of D-gluconate to 2-dehydro-D-gluconate is catalyzed by GADH, which is integral to the metabolism of gluconic acid derivatives. This reaction is essential for the production of other valuable compounds, including:
- 2,5-Diketo-D-gluconate : A precursor for synthesizing L-ascorbic acid.
- L-Idonate : Formed through further reduction of this compound .
Table 1: Enzymatic Reactions Involving this compound
Reaction | Enzyme | Products |
---|---|---|
D-Gluconate + NADP+ → this compound + NADPH | GADH | This compound |
This compound + NADP+ → 2,5-Diketo-D-Gluconate + NADPH | Unknown | 2,5-Diketo-D-Gluconate |
This compound + NADP+ → L-Idonate + NADPH | Unknown | L-Idonate |
Microbial Metabolism
Research has highlighted the role of various microorganisms in the production of this compound. For instance, Gluconobacter species are known for their ability to oxidize D-glucose to gluconic acid and subsequently to this compound . This metabolic pathway is utilized in biotechnological applications for producing organic acids and other metabolites.
Bioelectrocatalysis
Recent studies have explored the use of this compound in bioelectrocatalytic systems. The direct electron transfer reactions involving GADH have been investigated to enhance the efficiency of biofuel cells. These systems utilize the oxidation of D-gluconate to generate electrical energy, showcasing the potential for sustainable energy applications .
Production via Microbial Fermentation
A study demonstrated that Gluconobacter sphaericus could be engineered for hyperproduction of this compound from glucose substrates. The researchers identified optimal fermentation conditions that led to significant yields, indicating its potential for industrial applications in organic acid production .
Applications in Food Industry
Due to its presence in natural products like honey and wine, this compound has implications in food science as a flavoring agent and preservative. Its antioxidant properties may contribute to food preservation and enhancement .
Q & A
Basic Research Questions
Q. What experimental methods are recommended for distinguishing 2-dehydro-D-gluconate from structurally similar metabolites (e.g., 2,5-didehydro-D-gluconate) in microbial extracts?
- Methodological Answer : Use high-performance liquid chromatography (HPLC) coupled with UV-Vis detection at 260 nm, as this compound absorbs strongly in this range due to its α-keto acid moiety. Confirm identity via enzymatic assays with purified gluconate 2-dehydrogenase (EC 1.1.99.3), which specifically oxidizes D-gluconate to this compound . Cross-validate with mass spectrometry (MS) or nuclear magnetic resonance (NMR) for unambiguous structural confirmation .
Q. How can researchers confirm the metabolic role of this compound in bacterial ketogluconate pathways?
- Methodological Answer : Employ isotopic tracing with -labeled glucose to track carbon flow into this compound via gluconate dehydrogenases (e.g., EC 1.1.1.215 or EC 1.1.99.3). Monitor intermediate accumulation in knockout strains lacking downstream enzymes like dehydrogluconate dehydrogenase (EC 1.1.99.4). Pair this with transcriptomic analysis to correlate enzyme expression levels with pathway activity .
Q. What are the optimal conditions for in vitro enzymatic synthesis of this compound?
- Methodological Answer : Use purified gluconate 2-dehydrogenase (EC 1.1.99.3) in a reaction buffer (pH 7.5–8.0) containing 50 mM Tris-HCl, 5 mM Mg, and 1 mM NADP. Maintain substrate (D-gluconate) concentrations at 10–20 mM to avoid substrate inhibition. Monitor reaction progress at 340 nm (NADPH formation) and terminate at 80% conversion to minimize product degradation .
Advanced Research Questions
Q. How can conflicting data on the reversibility of this compound dehydrogenase (EC 1.1.99.4) in vivo versus in vitro be resolved?
- Methodological Answer : Perform thermodynamic profiling using isothermal titration calorimetry (ITC) to measure equilibrium constants under physiological conditions (e.g., pH, redox potential). Compare in vitro activity with in vivo flux analysis via -metabolic flux analysis (MFA) in Agrobacterium tumefaciens or H. pylori models. Address discrepancies by evaluating enzyme regulation (e.g., allosteric effectors, post-translational modifications) that may alter directionality .
Q. What strategies are effective for resolving ambiguities in computational predictions of this compound-associated pathways (e.g., false-positive annotations)?
- Methodological Answer : Integrate pathway-tools like KEGG or MetaCyc with manual curation using evidence codes (e.g., genomic context, gene co-expression). Validate predicted pathways by knocking out candidate genes (e.g., gntK or kguD) and analyzing metabolite accumulation via LC-MS. Cross-reference with phylogenomic databases to ensure enzyme specificity, as misannotation of gluconate dehydrogenases is common .
Q. How can researchers address the lack of structural data for this compound-binding enzymes to improve catalytic engineering?
- Methodological Answer : Use homology modeling (e.g., SWISS-MODEL) based on templates like gluconate dehydrogenase (PDB: 2J7X) or α-ketoglutarate dehydrogenase. Validate binding pocket predictions via site-directed mutagenesis of conserved residues (e.g., Arg273 in EC 1.1.99.4). Pair with molecular dynamics simulations to assess substrate docking stability. Experimental validation via X-ray crystallography or cryo-EM is critical for high-confidence models .
Q. Key Methodological Notes
- Enzymatic Assays : Standardize activity measurements using protocols from Shinagawa et al. (1976) for EC 1.1.99.4, monitoring acceptor-specific reduction rates .
- Data Contradictions : Resolve pathway conflicts by integrating multi-omics (proteomics, metabolomics) and genetic perturbation data .
- Computational Tools : Leverage platforms like PathPred or RetroPathRL for pathway hypothesis generation, but prioritize manual curation for accuracy .
Comparison with Similar Compounds
Structural Isomers and Derivatives
Table 1: Structural Comparison of 2-Dehydro-D-gluconate and Related Compounds
Key Observations :
- Positional Isomers : The keto group’s position (C2 vs. C5) dictates enzyme specificity. For example, gluconate 2-dehydrogenase (EC 1.1.99.3) exclusively oxidizes D-gluconate to this compound , whereas 5-dehydro-D-gluconate requires distinct enzymes .
- Phosphorylated Derivatives : 6-Phospho-2-dehydro-D-gluconate is central to the ED pathway in bacteria like Pseudomonas putida, enabling ATP production without glycolysis .
- Deoxy Variants : 3-Dehydro-2-deoxy-D-gluconate lacks a hydroxyl group at C2, altering its reactivity and resistance to certain enzymatic steps .
Metabolic Pathway Roles
Table 2: Pathway-Specific Roles of this compound and Analogues
Key Findings :
- PPP vs. Glycolysis Crosstalk : In Blattella germanica (cockroach fat body), abamectin exposure increased this compound (PPP) while reducing 3-PGA (glycolysis), indicating metabolic rerouting for ATP production .
- Microbial Specificity: Citrobacter europaeus metabolizes this compound to produce acid, whereas Cypionkella collinsensis cannot, highlighting species-dependent utilization .
Key Insights :
- Fertility Biomarker : Elevated this compound in cattle plasma suggests PPP imbalance may impair reproductive success, unlike lactate or acetate, which reflect general energy status .
- Decomposition Ecology: this compound’s correlation with Pseudomonas abundance underscores its niche role in postmortem nutrient cycling, distinct from acetylated amino acids .
Properties
IUPAC Name |
(3S,4R,5R)-3,4,5,6-tetrahydroxy-2-oxohexanoate | |
---|---|---|
Details | Computed by LexiChem 2.6.6 (PubChem release 2019.06.18) | |
Source | PubChem | |
URL | https://pubchem.ncbi.nlm.nih.gov | |
Description | Data deposited in or computed by PubChem | |
InChI |
InChI=1S/C6H10O7/c7-1-2(8)3(9)4(10)5(11)6(12)13/h2-4,7-10H,1H2,(H,12,13)/p-1/t2-,3-,4+/m1/s1 | |
Details | Computed by InChI 1.0.5 (PubChem release 2019.06.18) | |
Source | PubChem | |
URL | https://pubchem.ncbi.nlm.nih.gov | |
Description | Data deposited in or computed by PubChem | |
InChI Key |
VBUYCZFBVCCYFD-JJYYJPOSSA-M | |
Details | Computed by InChI 1.0.5 (PubChem release 2019.06.18) | |
Source | PubChem | |
URL | https://pubchem.ncbi.nlm.nih.gov | |
Description | Data deposited in or computed by PubChem | |
Canonical SMILES |
C(C(C(C(C(=O)C(=O)[O-])O)O)O)O | |
Details | Computed by OEChem 2.1.5 (PubChem release 2019.06.18) | |
Source | PubChem | |
URL | https://pubchem.ncbi.nlm.nih.gov | |
Description | Data deposited in or computed by PubChem | |
Isomeric SMILES |
C([C@H]([C@H]([C@@H](C(=O)C(=O)[O-])O)O)O)O | |
Details | Computed by OEChem 2.1.5 (PubChem release 2019.06.18) | |
Source | PubChem | |
URL | https://pubchem.ncbi.nlm.nih.gov | |
Description | Data deposited in or computed by PubChem | |
Molecular Formula |
C6H9O7- | |
Details | Computed by PubChem 2.1 (PubChem release 2019.06.18) | |
Source | PubChem | |
URL | https://pubchem.ncbi.nlm.nih.gov | |
Description | Data deposited in or computed by PubChem | |
Molecular Weight |
193.13 g/mol | |
Details | Computed by PubChem 2.1 (PubChem release 2021.05.07) | |
Source | PubChem | |
URL | https://pubchem.ncbi.nlm.nih.gov | |
Description | Data deposited in or computed by PubChem | |
Retrosynthesis Analysis
AI-Powered Synthesis Planning: Our tool employs the Template_relevance Pistachio, Template_relevance Bkms_metabolic, Template_relevance Pistachio_ringbreaker, Template_relevance Reaxys, Template_relevance Reaxys_biocatalysis model, leveraging a vast database of chemical reactions to predict feasible synthetic routes.
One-Step Synthesis Focus: Specifically designed for one-step synthesis, it provides concise and direct routes for your target compounds, streamlining the synthesis process.
Accurate Predictions: Utilizing the extensive PISTACHIO, BKMS_METABOLIC, PISTACHIO_RINGBREAKER, REAXYS, REAXYS_BIOCATALYSIS database, our tool offers high-accuracy predictions, reflecting the latest in chemical research and data.
Strategy Settings
Precursor scoring | Relevance Heuristic |
---|---|
Min. plausibility | 0.01 |
Model | Template_relevance |
Template Set | Pistachio/Bkms_metabolic/Pistachio_ringbreaker/Reaxys/Reaxys_biocatalysis |
Top-N result to add to graph | 6 |
Feasible Synthetic Routes
Disclaimer and Information on In-Vitro Research Products
Please be aware that all articles and product information presented on BenchChem are intended solely for informational purposes. The products available for purchase on BenchChem are specifically designed for in-vitro studies, which are conducted outside of living organisms. In-vitro studies, derived from the Latin term "in glass," involve experiments performed in controlled laboratory settings using cells or tissues. It is important to note that these products are not categorized as medicines or drugs, and they have not received approval from the FDA for the prevention, treatment, or cure of any medical condition, ailment, or disease. We must emphasize that any form of bodily introduction of these products into humans or animals is strictly prohibited by law. It is essential to adhere to these guidelines to ensure compliance with legal and ethical standards in research and experimentation.