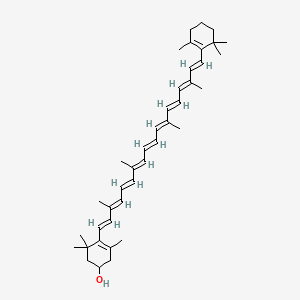
Cryptoxanthin, (+/-)-
- Click on QUICK INQUIRY to receive a quote from our team of experts.
- With the quality product at a COMPETITIVE price, you can focus more on your research.
Overview
Description
(±)-Cryptoxanthin, commonly referred to as β-cryptoxanthin, is a oxygenated carotenoid with the molecular formula C₄₀H₅₆O and molecular weight 552.85 g/mol . It exists in both free and esterified forms in nature, predominantly found in citrus fruits, red peppers, and pumpkin . Structurally, it features a β-ionone ring with a hydroxyl group at position 3, enabling provitamin A activity and antioxidant properties . Unlike non-polar carotenoids like β-carotene, β-cryptoxanthin's hydroxyl group enhances its solubility in lipid membranes, influencing its bioavailability and biological activity .
Preparation Methods
Synthetic Routes and Reaction Conditions: Cryptoxanthin can be synthesized through various methods, including the extraction from natural sources and chemical synthesis. The extraction process typically involves the use of solvents such as ethanol and hexane to isolate the carotenoid from plant materials . The chemical synthesis of cryptoxanthin involves the use of high-performance liquid chromatography (HPLC) to separate and purify the compound from other carotenoids .
Industrial Production Methods: In industrial settings, cryptoxanthin is often produced through the extraction from fruits and vegetables that are rich in this carotenoid. The extraction process is optimized to ensure high yield and purity, using techniques such as solvent extraction and HPLC . The extracted cryptoxanthin is then purified and processed for use in various applications, including as a food additive and nutritional supplement .
Chemical Reactions Analysis
Metabolic Pathways and Enzymatic Cleavage
Esterification with Fatty Acids
In citrus fruits, β-cryptoxanthin forms esters with fatty acids (e.g., laurate, myristate, palmitate ). These esters account for >90% of total β-cryptoxanthin in mature fruits . Esterification occurs during ripening, enhancing stability by preventing enzymatic cleavage. For example, CitCCD1 and CitCCD4 cleave free β-cryptoxanthin but not its esters .
Bioavailability and Absorption
β-Cryptoxanthin exhibits higher bioavailability than β-carotene due to its hydrophilicity and preferential absorption via SR-B1 receptors . Its localization on micelle surfaces during digestion enhances solubility and absorption .
Oxidative Stability and Antioxidant Activity
β-Cryptoxanthin reduces oxidative stress by scavenging lipid peroxides and suppressing pro-inflammatory cytokines (e.g., TNF-α, IL-6) in non-alcoholic fatty liver disease (NAFLD) models . While less potent than lycopene in singlet oxygen quenching, its antioxidant activity contributes to biological effects .
Structural Considerations
The crystalline structure of β-cryptoxanthin in plant chromoplasts influences its reactivity. Esterification with fatty acids alters its solubility and enzymatic accessibility, as seen in citrus flavedo and juice sacs .
Scientific Research Applications
Health Benefits and Mechanisms of Action
- Antioxidant Activity :
-
Prevention of Non-Alcoholic Fatty Liver Disease (NAFLD) :
- Recent studies have shown that β-cryptoxanthin can improve insulin resistance and reduce inflammation in models of NAFLD. It has been demonstrated to inhibit hepatic steatosis (fat accumulation in the liver) through mechanisms involving the modulation of lipid metabolism pathways, including activation of peroxisome proliferator-activated receptors (PPARs) .
- Bone Health :
- Anti-Inflammatory Effects :
Study 1: Effects on Hepatic Steatosis
A study involving β-cryptoxanthin supplementation in Bco2 -/- knockout mice demonstrated significant reductions in hepatic triglyceride levels and inflammation. The beneficial effects were attributed to increased activity of SIRT1 deacetylase, which modulates lipid metabolism by decreasing lipogenesis and enhancing fatty acid oxidation .
Study 2: Bone Health Implications
Research indicated that diets rich in β-cryptoxanthin correlate with higher bone density among older adults. The mechanism proposed involves its antioxidant properties that protect osteoblasts from oxidative damage, enhancing bone formation processes .
Comparative Data Table
Mechanism of Action
The mechanism of action of cryptoxanthin involves its conversion to vitamin A (retinol) in the human body. This conversion is facilitated by the enzyme β-carotene 15,15’-monooxygenase, which cleaves cryptoxanthin to form retinal . Retinal is then further converted to retinol, which is essential for vision, immune function, and cellular growth . Additionally, cryptoxanthin acts as an antioxidant, helping to neutralize free radicals and prevent oxidative damage to cells and DNA .
Comparison with Similar Compounds
Comparative Analysis with Structurally Similar Compounds
α-Cryptoxanthin
- Structural Differences: α-Cryptoxanthin (zeinoxanthin) has a β,ε-carotene-3-ol structure, differing from β-cryptoxanthin’s β,β-carotene-3-ol configuration. This structural variance reduces its provitamin A activity compared to β-cryptoxanthin .
- Bioavailability : In female Wistar rats, α-cryptoxanthin exhibited 14% lower absorption efficiency than β-cryptoxanthin, likely due to differences in intestinal uptake mechanisms .
- Dietary Sources : Found in smaller quantities in corn and certain citrus varieties .
β-Carotene
- Provitamin A Activity : β-Cryptoxanthin has ~50% of β-carotene’s vitamin A activity but is more bioavailable in rodents and humans .
- Antioxidant Capacity : β-Cryptoxanthin demonstrates superior singlet oxygen quenching compared to β-carotene and vitamin E, attributed to its conjugated double-bond system .
- Health Impacts : Unlike β-carotene, β-cryptoxanthin uniquely inhibits bone resorption by suppressing parathyroid hormone (PTH)-induced osteoclast activation .
Lutein and Zeaxanthin
- Polarity and Localization: Lutein and zeaxanthin are dihydroxy carotenoids, making them more polar than β-cryptoxanthin. This polarity directs their accumulation in the macula lutea of the eye, whereas β-cryptoxanthin is more concentrated in the liver .
- Health Roles : While lutein/zeaxanthin are critical for macular pigment density, β-cryptoxanthin is inversely associated with advanced age-related macular degeneration (AMD) risk, suggesting a complementary protective role .
Astaxanthin
- Antioxidant Potency: Astaxanthin, a keto-carotenoid, has a higher antioxidant capacity due to additional ketone groups. However, β-cryptoxanthin is more effective in reducing hepatic lipid peroxidation in non-alcoholic steatohepatitis (NASH) models .
- Therapeutic Applications : β-Cryptoxanthin reduced hepatic triglycerides (TG) by 30–40% in mice fed a high-fat diet, outperforming astaxanthin in mitigating mitochondrial dysfunction .
Pharmacokinetic and Functional Comparisons
Bioavailability and Metabolism
Health Outcomes
- Cancer Risk : Low serum β-cryptoxanthin correlated with a 2.7-fold higher cervical cancer risk , comparable to β-carotene’s protective effects .
- Hepatic Benefits : In NASH models, β-cryptoxanthin reduced hepatic TG by 50% and suppressed pro-inflammatory cytokines (TNF-α, IL-6) more effectively than vitamin E .
Biological Activity
Cryptoxanthin, specifically β-cryptoxanthin, is a carotenoid known for its potent biological activities, including antioxidant properties and potential health benefits. This article provides a comprehensive overview of the biological activity of β-cryptoxanthin, focusing on its mechanisms of action, effects on various health conditions, and relevant case studies.
1. Overview of β-Cryptoxanthin
β-Cryptoxanthin is a xanthophyll carotenoid predominantly found in fruits such as Satsuma mandarin oranges and papayas. It serves as a provitamin A compound and exhibits significant antioxidant activity, which is crucial for mitigating oxidative stress in the body.
β-Cryptoxanthin exerts its biological effects through several mechanisms:
- Antioxidant Activity : It scavenges free radicals, thereby reducing oxidative stress and inflammation .
- Gene Expression Modulation : It influences the expression of genes related to lipid metabolism and cell cycle regulation, particularly in cancer cells .
- Bone Health : β-Cryptoxanthin stimulates osteoblastic activity and inhibits osteoclastic resorption, contributing to improved bone density and prevention of osteoporosis .
3.1 Cancer Prevention
Epidemiological studies suggest an inverse relationship between β-cryptoxanthin intake and lung cancer risk. Experimental studies have shown that it can inhibit the growth of non-small cell lung cancer cells by modulating cell cycle regulators such as cyclins and p21 .
3.2 Liver Health
Research indicates that β-cryptoxanthin supplementation can reduce hepatic lipid accumulation and inflammation in models of non-alcoholic fatty liver disease (NAFLD). It appears to operate independently of its conversion to retinoids, suggesting direct effects on liver metabolism .
3.3 Bone Metabolism
Studies demonstrate that β-cryptoxanthin enhances bone formation while inhibiting bone resorption. It promotes osteoblastic proliferation and mineralization, making it a candidate for osteoporosis prevention .
4.1 Liver Steatosis Study
A study involving Bco2 knockout mice showed that β-cryptoxanthin supplementation significantly lowered hepatic triglyceride levels after three months, indicating its protective role against liver steatosis .
4.2 Osteoporosis Prevention
In a clinical trial involving postmenopausal women, long-term intake of β-cryptoxanthin-rich juice was associated with increased bone mineral density, highlighting its potential as a dietary intervention for osteoporosis .
5. Tables of Research Findings
6. Conclusion
The biological activity of β-cryptoxanthin underscores its potential as a functional food component with health-promoting properties. Its multifaceted roles in cancer prevention, liver health, and bone metabolism make it an important subject for further research to fully elucidate its mechanisms and therapeutic applications.
Q & A
Basic Research Questions
Q. What analytical methods are recommended for quantifying cryptoxanthin isomers (e.g., free vs. esterified forms) in plant and biological samples?
To quantify cryptoxanthin isomers, high-performance liquid chromatography (HPLC) coupled with UV detection or mass spectrometry (MS) is the gold standard. For plant samples, the distribution of free and esterified forms depends on species and environmental factors, necessitating saponification steps to hydrolyze esters before analysis. Normal-phase HPLC effectively separates carotenoids like α- and β-cryptoxanthin, while reverse-phase systems are better suited for esterified forms . Validation using reference standards and internal controls (e.g., α-tocopherol) is critical to avoid spectral overlap .
Q. How does the bioavailability of cryptoxanthin compare to other carotenoids in human dietary studies?
β-Cryptoxanthin exhibits higher bioavailability than β-carotene and lycopene in humans, partly due to its efficient micellarization during digestion. Studies using canned citrus fruit meals demonstrated significant increases in plasma β-cryptoxanthin levels, whereas β-carotene and lycopene remained unchanged. Bioavailability is influenced by food matrix, lipid content, and individual metabolic factors, requiring crossover trial designs with standardized diets to control variables .
Q. What experimental models are suitable for studying cryptoxanthin’s antioxidant effects in vitro?
Cell lines such as HepG2 (liver) and Caco-2 (intestinal) are commonly used to assess antioxidant activity via assays like DPPH radical scavenging and lipid peroxidation inhibition. For mechanistic studies, gene expression profiling (e.g., Nrf2 pathway activation) and redox-sensitive fluorescent probes (e.g., DCFH-DA) provide quantitative data. Dose-response curves should account for cryptoxanthin’s biphasic effects, where low doses may act as antioxidants but high doses could induce pro-oxidant activity .
Advanced Research Questions
Q. How can researchers resolve contradictions in epidemiological data linking cryptoxanthin intake to cancer risk reduction, particularly in smokers?
Conflicting results (e.g., reduced lung cancer risk in smokers vs. null effects) arise from confounding variables like smoking intensity and genetic polymorphisms (e.g., CYP1A1). Mendelian randomization studies can mitigate bias by leveraging genetic variants as proxies for cryptoxanthin exposure. Additionally, stratified analyses by smoking status and adjustment for oxidative stress biomarkers (e.g., 8-OHdG) are essential. Meta-analyses should prioritize cohort studies over case-control designs to minimize recall bias .
Q. What molecular mechanisms underlie cryptoxanthin’s dual role in cell cycle arrest and apoptosis in leukemia models?
In K-562 leukemia cells, cryptoxanthin induces G2/M arrest via tubulin polymerization disruption, while caspase-3/9 activation drives apoptosis in CRL-2724 cells. Mechanistic studies require flow cytometry for cell cycle profiling and Western blotting for apoptosis markers (e.g., PARP cleavage). Differential effects may stem from BCR-ABL fusion gene expression in K-562, necessitating siRNA knockdown experiments to validate pathway specificity .
Q. How does cryptoxanthin modulate macrophage polarization in non-alcoholic steatohepatitis (NASH) models, and what are the implications for experimental design?
Cryptoxanthin promotes M2 macrophage polarization via IL-4/STAT6 signaling, reducing hepatic inflammation in NASH. Experimental workflows should include:
- In vitro : Bone marrow-derived macrophages treated with cryptoxanthin and LPS/IFN-γ (M1) or IL-4 (M2), followed by qPCR for Arg1 and iNOS.
- In vivo : C57BL/6J mice fed a choline-deficient, L-amino acid-defined diet, with hepatic gene expression analyzed via RNA-seq and Ingenuity Pathway Analysis (IPA).
Tissue-specific accumulation of cryptoxanthin in the liver must be confirmed via HPLC .
Q. What strategies are recommended for reconciling discrepancies in cryptoxanthin’s effects across in vitro and in vivo cancer models?
Discrepancies often arise from differences in metabolite formation (e.g., retinol vs. intact cryptoxanthin) and tumor microenvironment interactions. Orthotopic xenograft models, where cancer cells are implanted in organ-matched sites, improve translational relevance. Pharmacokinetic studies measuring cryptoxanthin and its metabolites in plasma and tissues are critical. Co-culture systems incorporating stromal cells (e.g., fibroblasts) can mimic in vivo conditions .
Q. Methodological Guidance
Q. How should researchers design animal studies to evaluate cryptoxanthin’s anti-inflammatory effects without confounding dietary variables?
- Use pair-fed controls to ensure caloric intake equivalence.
- Administer cryptoxanthin via oral gavage with lipid vehicles (e.g., olive oil) to standardize absorption.
- Measure hepatic and serum cryptoxanthin levels post-sacrifice using HPLC, normalized to organ weight.
- Include endpoints like plasma TNF-α and liver histopathology (e.g., H&E staining for inflammatory foci) .
Q. What statistical approaches are optimal for analyzing cryptoxanthin’s dose-dependent effects in heterogeneous cell populations?
Non-linear regression models (e.g., sigmoidal dose-response) with bootstrapping are preferred over ANOVA for dose-response data. For single-cell heterogeneity, flow cytometry combined with t-SNE clustering can identify subpopulations with divergent cryptoxanthin sensitivity. Bayesian hierarchical models account for batch effects in multi-laboratory studies .
Q. How can researchers address the instability of cryptoxanthin during sample storage and processing?
Properties
CAS No. |
31272-51-2 |
---|---|
Molecular Formula |
C40H56O |
Molecular Weight |
552.9 g/mol |
IUPAC Name |
3,5,5-trimethyl-4-[(1E,3E,5E,7E,9E,11E,13E,15E,17E)-3,7,12,16-tetramethyl-18-(2,6,6-trimethylcyclohexen-1-yl)octadeca-1,3,5,7,9,11,13,15,17-nonaenyl]cyclohex-3-en-1-ol |
InChI |
InChI=1S/C40H56O/c1-30(18-13-20-32(3)23-25-37-34(5)22-15-27-39(37,7)8)16-11-12-17-31(2)19-14-21-33(4)24-26-38-35(6)28-36(41)29-40(38,9)10/h11-14,16-21,23-26,36,41H,15,22,27-29H2,1-10H3/b12-11+,18-13+,19-14+,25-23+,26-24+,30-16+,31-17+,32-20+,33-21+ |
InChI Key |
DMASLKHVQRHNES-QQGJMDNJSA-N |
Isomeric SMILES |
CC1=C(C(CCC1)(C)C)/C=C/C(=C/C=C/C(=C/C=C/C=C(\C)/C=C/C=C(\C)/C=C/C2=C(CC(CC2(C)C)O)C)/C)/C |
Canonical SMILES |
CC1=C(C(CCC1)(C)C)C=CC(=CC=CC(=CC=CC=C(C)C=CC=C(C)C=CC2=C(CC(CC2(C)C)O)C)C)C |
Origin of Product |
United States |
Disclaimer and Information on In-Vitro Research Products
Please be aware that all articles and product information presented on BenchChem are intended solely for informational purposes. The products available for purchase on BenchChem are specifically designed for in-vitro studies, which are conducted outside of living organisms. In-vitro studies, derived from the Latin term "in glass," involve experiments performed in controlled laboratory settings using cells or tissues. It is important to note that these products are not categorized as medicines or drugs, and they have not received approval from the FDA for the prevention, treatment, or cure of any medical condition, ailment, or disease. We must emphasize that any form of bodily introduction of these products into humans or animals is strictly prohibited by law. It is essential to adhere to these guidelines to ensure compliance with legal and ethical standards in research and experimentation.