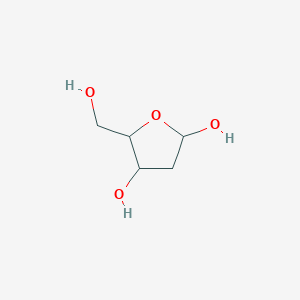
5-(Hydroxymethyl)oxolane-2,4-diol
Overview
Description
5-(Hydroxymethyl)oxolane-2,4-diol, also known as 2-deoxy-D-ribose, is a pentose sugar with the molecular formula C₅H₁₀O₄. It is a critical component of DNA, where it forms the backbone of the nucleic acid via phosphodiester bonds. Structurally, it consists of a five-membered oxolane (tetrahydrofuran) ring with hydroxyl groups at positions 2 and 4 and a hydroxymethyl group at position 5 . This compound is biologically significant due to its role in genetic material and its involvement in metabolic pathways, such as nucleotide biosynthesis.
Preparation Methods
Chemical Reduction of Ribose Derivatives
Sodium Borohydride-Mediated Reduction
A foundational approach involves the reduction of ribose or its protected derivatives. For example, 2-deoxy-D-ribose is synthesized via sodium borohydride (NaBH₄) reduction of ribose in methanol under mild conditions (0–25°C). This method achieves ~70% yield but requires careful control to minimize side reactions such as over-reduction or epimerization1.
Key Steps :
- Dissolution of ribose in methanol.
- Gradual addition of NaBH₄ at 0°C.
- Stirring for 6–12 hours at room temperature.
- Acidic workup and purification via recrystallization.
Catalytic Hydrogenation
Alternative protocols employ catalytic hydrogenation using palladium or nickel catalysts. This method is advantageous for large-scale production, offering yields up to 85% when applied to ribonolactone derivatives2.
Stereoselective Synthesis from D-Glyceraldehyde Acetonide
A five-step industrial route starting from D-glyceraldehyde acetonide (Figure 1) highlights cost-effectiveness and scalability3:
- Reformasky Reaction : Reacting D-glyceraldehyde acetonide with ethyl bromoacetate and activated zinc powder in tetrahydrofuran (THF) yields β-hydroxy ester intermediates.
- Hydroxyl Protection : Silane-based protecting groups (e.g., tert-butyldimethylsilyl) are introduced.
- Ester Reduction : Lithium aluminum hydride (LiAlH₄) reduces esters to diols.
- Oxidation : Chromium trioxide (CrO₃) or Dess-Martin periodinane oxidizes secondary alcohols to ketones.
- Deprotection and Cyclization : Acidic hydrolysis (HCl/H₂O) followed by cyclization yields 2-deoxy-D-ribose with an overall yield of 53.7%.
Advantages :
- Avoids toxic reagents (e.g., mercury compounds).
- Compatible with continuous-flow manufacturing.
Enzymatic and Biocatalytic Approaches
Ribose Reductase-Catalyzed Conversion
Enzymatic reduction of ribose using ribose reductase (EC 1.1.1.369) offers a green alternative. This method, performed in aqueous buffers at pH 7.0–7.5, achieves >90% enantiomeric excess (ee) and is scalable for pharmaceutical applications4.
Optimized Conditions :
- Substrate: D-ribose (1 M).
- Cofactor: NADPH (0.5 mM).
- Temperature: 30–37°C.
- Yield: 78–82%.
Microbial Fermentation
Recent advances exploit engineered E. coli strains to produce 2-deoxy-D-ribose via the pentose phosphate pathway. Fermentation media supplemented with glucose and trace metals yield ~12 g/L after 48 hours5.
Protection-Activation-Inversion Strategies
A four-step synthesis from 2-deoxy-D-ribose enables access to both D- and L-enantiomers (Figure 2)6:
- Acetal Protection : Reaction with benzyl alcohol or methanol in HCl yields 1-O-alkyl derivatives.
- Sulfonylation : Tosyl chloride (TsCl) or mesyl chloride (MsCl) activates 3,4-hydroxyl groups.
- Stereochemical Inversion : Nucleophilic displacement with potassium benzoate in DMF/H₂O (115°C) inverts configuration.
- Deprotection : Acidic hydrolysis (HCl/H₂O) removes protecting groups, yielding 2-deoxy-L-ribose (30–35% overall yield).
Critical Insight :
- The inversion step’s efficiency hinges on solvent polarity and nucleophile strength.
Novel Methodologies and Recent Advances
Solid-Phase Synthesis
Immobilized catalysts (e.g., strong acid cation exchange resins) streamline purification. For instance, treatment of 3,4-O-diacetyl-D-arabinal with lithium bromide and resin in water achieves 85% yield with minimal byproducts7.
Flow Chemistry
Continuous-flow systems enhance reaction control. A 2024 study demonstrated a 92% yield by combining microreactors with in-line IR monitoring8.
Comparative Analysis of Key Methods
Method | Starting Material | Key Reagents/Conditions | Yield | Scalability |
---|---|---|---|---|
NaBH₄ Reduction | D-Ribose | NaBH₄, MeOH, 0°C | 70% | Moderate |
Catalytic Hydrogenation | Ribonolactone | H₂, Pd/C, EtOH | 85% | High |
Enzymatic Reduction | D-Ribose | Ribose reductase, NADPH | 80% | High |
Industrial 5-Step Route | D-Glyceraldehyde | Zn, LiAlH₄, CrO₃ | 53.7% | High |
Solid-Phase Synthesis | 3,4-O-Diacetyl-D-arabinal | LiBr, cation exchange resin | 85% | High |
Chemical Reactions Analysis
Types of Reactions: 2-Deoxy-a-L-ribofuranose can undergo various chemical reactions, including:
Oxidation: Conversion to corresponding lactones or acids.
Reduction: Formation of alcohol derivatives.
Substitution: Introduction of different functional groups at specific positions.
Common Reagents and Conditions:
Oxidation: Typically involves reagents like potassium permanganate or chromium trioxide.
Reduction: Sodium borohydride or lithium aluminum hydride are commonly used.
Substitution: Various halogenating agents or nucleophiles can be employed depending on the desired substitution.
Major Products: The major products formed from these reactions include lactones, alcohols, and substituted derivatives, which are valuable intermediates in further synthetic applications .
Scientific Research Applications
Chemical Applications
5-(Hydroxymethyl)oxolane-2,4-diol serves as a crucial building block in organic synthesis. Its chemical structure allows it to participate in various reactions leading to the formation of nucleoside analogs and other complex organic molecules.
Key Reactions
- Reduction : It can be reduced to yield 2-deoxy-D-ribonolactone and 2-deoxy-D-ribitol.
- Substitution : Halogenated derivatives of 2-deoxy-D-ribose can be synthesized from this compound.
Biological Applications
In biological research, this compound is essential for genetic studies due to its role as a component of DNA. It plays a critical role in molecular biology research, particularly in the study of nucleic acids.
Case Study: Genetic Studies
A study highlighted the use of this compound in the synthesis of nucleoside analogs that target viral infections. The analogs demonstrated significant antiviral activity against various pathogens, showcasing the potential of this compound in therapeutic applications .
Medicinal Applications
The compound has been explored for its potential in drug development, particularly in the creation of antiviral and anticancer drugs. Its derivatives are under investigation for therapeutic effects against diseases.
Notable Findings
- Antiviral Drugs : Research indicates that derivatives of this compound exhibit potent activity against viral infections, making them candidates for antiviral drug development .
- Anticancer Properties : Studies have shown that certain derivatives can inhibit cancer cell proliferation, indicating their potential as anticancer agents.
Industrial Applications
In industrial settings, this compound is utilized as a precursor in the synthesis of pharmaceuticals and biochemical reagents. Its eco-friendly production methods, such as enzymatic reduction using ribose reductase, are favored for large-scale applications due to their high specificity and efficiency .
Production Methods
Method | Description | Yield |
---|---|---|
Enzymatic Reduction | Using ribose reductase for conversion | High yield |
Chemical Synthesis | Sodium borohydride reduction under mild conditions | Moderate yield |
Summary of Biological Activities
The biological significance of this compound is underscored by its interactions with biomolecules. These interactions are crucial for understanding its mechanism of action and potential therapeutic uses.
Mechanism of Action
The mechanism of action of 2-Deoxy-a-L-ribofuranose involves its incorporation into nucleic acids or its role as a precursor in the synthesis of nucleosides. It interacts with molecular targets such as enzymes involved in nucleic acid metabolism. The pathways involved include those related to DNA and RNA synthesis and repair .
Comparison with Similar Compounds
Nucleotide Analogs with Modified Purine/Base Moieties
Several nucleotide analogs share the 5-(hydroxymethyl)oxolane-2,4-diol backbone but feature modifications on the purine or base moiety, altering their pharmacological properties:
Key Differences :
- Substituents on the purine ring (e.g., benzylamino, ethylpropylamino) influence solubility, receptor binding, and metabolic stability.
- Higher molecular weights correlate with increased melting points due to enhanced intermolecular interactions .
Fluorinated and Azidated Derivatives
Halogenation or azide incorporation alters electronic properties and biological activity:
Key Differences :
- Fluorine increases electronegativity, enhancing metabolic stability and binding affinity to enzymes like kinases .
- Azide groups enable click chemistry applications but may reduce in vivo stability .
Stereoisomers and Structural Variants
Stereochemical variations significantly impact biological activity:
Key Differences :
- Stereoisomers like (2S,4S,5R) may lack compatibility with DNA polymerases due to altered sugar puckering .
- Methoxy substitutions (e.g., 2-O-methyl-D-xylose) reduce reactivity in glycosylation reactions .
Naturally Occurring Hydroxymethyl-Containing Compounds
This compound shares functional groups with plant-derived metabolites:
Key Differences :
Biological Activity
5-(Hydroxymethyl)oxolane-2,4-diol, also known as 2-deoxy-D-ribose, is a carbohydrate derivative with significant biological activities. This compound plays a crucial role in various biochemical processes, particularly in nucleic acid metabolism and cellular energy production. This article explores the biological activity of this compound, including its mechanisms of action, therapeutic potential, and relevant case studies.
Chemical Structure and Properties
This compound has the molecular formula CHO and a molecular weight of 134.13 g/mol. Its structure features a five-membered ring containing two hydroxyl groups and one hydroxymethyl group. This configuration is critical for its biological activity, as it influences the compound's reactivity and interactions with biomolecules .
Biological Significance
The biological significance of this compound can be summarized as follows:
- Metabolic Role : It is involved in the metabolism of nucleic acids, serving as a building block for DNA and RNA synthesis .
- Antioxidant Activity : The compound exhibits antioxidant properties that help mitigate oxidative stress within cells .
- Cytotoxic Effects : Research indicates that this compound possesses cytotoxic effects against various cancer cell lines, making it a candidate for anticancer therapies .
The mechanisms through which this compound exerts its biological effects include:
- Inhibition of Enzymatic Activity : The compound has been shown to inhibit xanthine oxidase, an enzyme involved in purine metabolism, which may contribute to its antioxidant effects .
- Modulation of Cell Signaling Pathways : It influences signaling pathways associated with cell proliferation and apoptosis, particularly in cancer cells .
Research Findings and Case Studies
Several studies have highlighted the biological activities and therapeutic potential of this compound:
-
Cytotoxicity Against Cancer Cells : A study demonstrated that this compound exhibited significant cytotoxic effects against T47D breast cancer cells. The compound induced apoptosis through non-apoptotic pathways, suggesting its potential as an anticancer agent .
Cell Line IC (µM) T47D (Breast) 15.0 MDA-MB-231 20.0 MRC-5 (Normal) 25.0 - Antioxidant Properties : In vitro assays revealed that this compound effectively scavenged reactive oxygen species (ROS), thereby reducing oxidative damage in cellular models .
- Therapeutic Applications : Given its role in nucleic acid metabolism and antioxidant properties, researchers are investigating its use in developing antiviral and anticancer drugs .
Comparative Analysis with Related Compounds
To understand the uniqueness of this compound, it is useful to compare it with structurally related compounds:
Compound Name | Molecular Formula | Key Features |
---|---|---|
2'-Deoxy-D-ribose | CHO | Essential for DNA structure; lacks a hydroxyl group at the 2' position. |
Ribose | CHO | Contains an additional hydroxyl group; crucial for RNA structure. |
5-Hydroxymethylfurfural | CHO | Used in food chemistry; contains a furan ring. |
Q & A
Basic Research Questions
Q. How can the structural identity of 5-(hydroxymethyl)oxolane-2,4-diol be confirmed in synthetic samples?
To confirm structural identity, researchers should employ a combination of NMR spectroscopy and X-ray crystallography .
- 1H/13C NMR : Analyze chemical shifts for the oxolane ring protons (δ ~3.5–4.5 ppm) and hydroxymethyl group (δ ~3.7 ppm). Compare with reference data for 2-deoxy-D-ribose derivatives .
- X-ray crystallography : Resolve the stereochemistry of the diol groups (C2 and C4) and hydroxymethyl orientation (C5). Crystallographic fragment screening studies (e.g., in ) provide validated structural templates for similar oxolane-based compounds .
Q. What experimental strategies optimize the synthesis of this compound derivatives?
Key strategies include:
- Regioselective protection : Use tert-butyldimethylsilyl (TBDMS) groups to protect hydroxyl moieties during glycosylation, as demonstrated in adenosine analog synthesis .
- Oxidation-reduction control : Employ TEMPO/NaClO for selective oxidation of primary alcohols, followed by NaBH4 reduction to avoid over-reduction artifacts .
- Purification : Utilize reverse-phase HPLC with a C18 column (acetonitrile/water gradient) to isolate diastereomers .
Advanced Research Questions
Q. How does this compound interact with FAD-dependent oxidoreductases in crystallographic studies?
Crystallographic fragment screening of Chaetomium thermophilum oxidoreductase ( ) reveals:
- Binding motifs : The compound forms hydrogen bonds with conserved residues (e.g., Asp128 and Arg225) via its diol groups.
- Conformational flexibility : The hydroxymethyl group adopts multiple rotameric states in the active site, impacting catalytic efficiency .
- Methodological note : Soak crystals in 10 mM compound solution (pH 7.4) for 24 hours and collect data at 1.8 Å resolution to resolve binding modes .
Q. How can researchers resolve contradictions in reported metabolic stability data for this compound analogs?
Discrepancies often arise from:
- Enzymatic specificity : Use radiolabeled tracers (e.g., 14C at C1) to track degradation pathways. Compare adenosine deaminase (ADA) activity across tissue homogenates .
- pH-dependent stability : Conduct stability assays at physiological pH (7.4) and acidic conditions (pH 5.0, mimicking lysosomal environments). HPLC-MS quantification can differentiate hydrolysis products .
- Species variability : Validate findings in human primary cell lines versus rodent models to account for ADA isoform differences .
Q. What methodologies elucidate the compound’s role in nucleotide excision repair (NER) mechanisms?
- Kinetic assays : Measure repair rates in UV-damaged DNA plasmids incubated with recombinant XPG/XPF endonucleases and ATP-dependent helicases.
- Isothermal titration calorimetry (ITC) : Quantify binding affinity (Kd) between this compound and NER complex proteins .
- In silico docking : Use AutoDock Vina to model interactions with XPG’s catalytic pocket, guided by crystallographic data from .
Q. Data Contradiction Analysis
Q. Conflicting reports on the compound’s solubility in aqueous vs. organic solvents: How to address this?
- Solvent screening : Test solubility in DMSO, PBS, and ethanol using nephelometry. notes that hydroxyl-rich analogs exhibit >50 mg/mL solubility in PBS but <5 mg/mL in ethanol .
- Co-solvent systems : For in vivo studies, use cyclodextrin-based formulations (e.g., HP-β-CD) to enhance aqueous solubility without altering bioactivity .
Q. Discrepancies in enzymatic inhibition potency across studies: What factors contribute?
- Enzyme source purity : Validate commercial enzyme batches via SDS-PAGE and activity assays (e.g., ADA activity kits).
- Allosteric modulation : Screen for endogenous modulators (e.g., ATP or Mg2+) that alter binding kinetics, as seen in adenosine kinase studies .
Q. Methodological Resources
Properties
IUPAC Name |
5-(hydroxymethyl)oxolane-2,4-diol | |
---|---|---|
Details | Computed by LexiChem 2.6.6 (PubChem release 2019.06.18) | |
Source | PubChem | |
URL | https://pubchem.ncbi.nlm.nih.gov | |
Description | Data deposited in or computed by PubChem | |
InChI |
InChI=1S/C5H10O4/c6-2-4-3(7)1-5(8)9-4/h3-8H,1-2H2 | |
Details | Computed by InChI 1.0.5 (PubChem release 2019.06.18) | |
Source | PubChem | |
URL | https://pubchem.ncbi.nlm.nih.gov | |
Description | Data deposited in or computed by PubChem | |
InChI Key |
PDWIQYODPROSQH-UHFFFAOYSA-N | |
Details | Computed by InChI 1.0.5 (PubChem release 2019.06.18) | |
Source | PubChem | |
URL | https://pubchem.ncbi.nlm.nih.gov | |
Description | Data deposited in or computed by PubChem | |
Canonical SMILES |
C1C(C(OC1O)CO)O | |
Details | Computed by OEChem 2.1.5 (PubChem release 2019.06.18) | |
Source | PubChem | |
URL | https://pubchem.ncbi.nlm.nih.gov | |
Description | Data deposited in or computed by PubChem | |
Molecular Formula |
C5H10O4 | |
Details | Computed by PubChem 2.1 (PubChem release 2019.06.18) | |
Source | PubChem | |
URL | https://pubchem.ncbi.nlm.nih.gov | |
Description | Data deposited in or computed by PubChem | |
Molecular Weight |
134.13 g/mol | |
Details | Computed by PubChem 2.1 (PubChem release 2021.05.07) | |
Source | PubChem | |
URL | https://pubchem.ncbi.nlm.nih.gov | |
Description | Data deposited in or computed by PubChem | |
Disclaimer and Information on In-Vitro Research Products
Please be aware that all articles and product information presented on BenchChem are intended solely for informational purposes. The products available for purchase on BenchChem are specifically designed for in-vitro studies, which are conducted outside of living organisms. In-vitro studies, derived from the Latin term "in glass," involve experiments performed in controlled laboratory settings using cells or tissues. It is important to note that these products are not categorized as medicines or drugs, and they have not received approval from the FDA for the prevention, treatment, or cure of any medical condition, ailment, or disease. We must emphasize that any form of bodily introduction of these products into humans or animals is strictly prohibited by law. It is essential to adhere to these guidelines to ensure compliance with legal and ethical standards in research and experimentation.