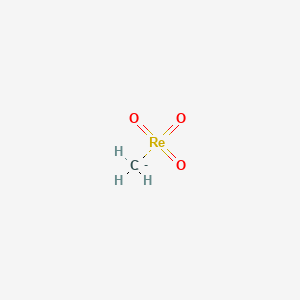
Methyltrioxorhenium(VII)
Overview
Description
Methyltrioxorhenium(VII) (CH₃ReO₃), commonly abbreviated as MTO, is a well-studied organorhenium compound characterized by a trigonal bipyramidal geometry with a methyl group and three oxygen atoms bonded to the rhenium center . First synthesized by Herrmann et al. in 1991, MTO has emerged as a versatile catalyst for oxidation reactions, particularly in the presence of hydrogen peroxide (H₂O₂) . Its catalytic prowess lies in its ability to activate H₂O₂, generating reactive peroxo intermediates (e.g., CH₃ReO(O₂)₂) that drive selective oxidation processes under mild conditions .
MTO exhibits exceptional activity in epoxidation, sulfoxidation, and hydroxylation reactions, with applications spanning pharmaceuticals, fragrances, and natural product synthesis . For example, it achieves turnover numbers (TONs) exceeding 20,000 in olefin epoxidation, outperforming most transition-metal catalysts . Its stability in acidic and aqueous environments further enhances its utility in industrial and laboratory settings .
Preparation Methods
Historical Evolution of MTO Synthesis
The first reported synthesis of MTO in 1979 involved the oxidation of trimethyldioxorhenium(VI) or tetramethyloxorhenium(VII) under dry air, yielding up to 50% MTO after weeks of exposure . This method’s inefficiency spurred innovations in organometallic reagent utilization. By the 1990s, protocols employing dirhenium heptoxide (Re₂O₇) with organozinc or organotin compounds became prevalent, though these introduced flammability and toxicity concerns . Contemporary approaches prioritize activating Re₂O₇ with silylation agents or carboxylic anhydrides to enhance reactivity while mitigating hazards .
Dirhenium Heptoxide-Based Methods
Organozinc Reagents
Reaction of Re₂O₇ with dimethylzinc (CH₃)₂Zn in anhydrous acetic acid produces MTO via the equation:
Yields reach 80–90%, but the method requires temperatures below −78°C to prevent Re(VII) reduction to lower oxidation states . Dimethylzinc’s pyrophoric nature necessitates inert atmospheres, complicating large-scale production. Post-reaction processing involves filtration to remove zinc oxides and distillation under vacuum, often necessitating sublimation for purity .
Organotin Reagents
Tetramethyltin (Sn(CH₃)₄) reacts with Re₂O₇ in acetonitrile at ambient temperature, yielding MTO and trimethyltin chloride:
This method achieves 70% yield but generates toxic tin byproducts, requiring silica gel filtration and solvent extraction for purification . Despite milder conditions, tetramethyltin’s neurotoxicity limits industrial adoption .
Silylation-Activated Routes
Trimethylsilyl Chloride (TMS-Cl) Mediated Synthesis
TMS-Cl activates Re₂O₇ by forming perrhenyl silyl esters, which react with organotin or organozinc reagents:
-
Silylation :
-
Organylation :
This two-step process converts all Re₂O₇ to MTO, avoiding stoichiometric losses . Yields improve to 80% with acetonitrile as the solvent, and silica gel filtration removes tin residues effectively .
Carboxylic Anhydride Activation
Acetic anhydride ((CH₃CO)₂O) reacts with Re₂O₇ to form perrhenyl acetate, which subsequently reacts with methylzinc carboxylates:
This method circumvents pyrophoric reagents but requires meticulous control of anhydrous conditions .
Comparative Analysis of Synthetic Methods
Key Observations :
-
Organozinc routes offer high yields but pose ignition risks.
-
Tin-based methods simplify temperature control but generate hazardous waste.
-
Silylation activation balances yield and safety but requires post-reaction purification .
Purification Techniques
Sublimation
Crude MTO sublimes at 40–60°C under vacuum (0.1 mmHg), yielding colorless crystals. While effective, scalability is limited by equipment costs and energy consumption .
Solvent Extraction and Filtration
Partitioning between dichloromethane and hexane removes nonpolar byproducts. Silica gel chromatography further eliminates tin or zinc residues, though it prolongs processing time .
Recrystallization
MTO recrystallizes from pentane or cyclohexane, enhancing purity to >95%. This method is preferred for small-scale laboratory synthesis .
Emerging Approaches and Innovations
Recent patents describe nontoxic routes using Re₂O₇ with nonpyrophoric organometallics (e.g., methylaluminum complexes) . Another advance involves solid-phase synthesis , where Re₂O₇ is immobilized on mesoporous silica, reducing reagent quantities and simplifying purification . These methods, however, remain experimental, with yields yet to match conventional approaches.
Chemical Reactions Analysis
Methyltrioxorhenium(VII) is known for its catalytic properties, especially in oxidation reactions. It activates hydrogen peroxide (H₂O₂) through an electrophilic mechanism, transferring a single oxygen atom to various substrates without the formation of free-radical intermediates . This makes it a highly efficient and selective catalyst.
Types of Reactions:
Substitution: It can also facilitate the substitution of hydrogen atoms in organic molecules with other functional groups under specific conditions.
Common Reagents and Conditions:
Oxidizing Agents: Hydrogen peroxide (H₂O₂) and urea hydrogen peroxide are commonly used in reactions involving methyltrioxorhenium(VII).
Major Products:
Epoxides: From the oxidation of alkenes.
Nitrones: From the oxidation of imines.
Carboxylic Acids/Esters: From the oxidation of terminal alkynes.
Scientific Research Applications
Oxidation Reactions
MTO is widely used as a catalyst for oxidation reactions, particularly in converting sulfoxides to sulfones using hydrogen peroxide. Research indicates that MTO facilitates these transformations effectively, with reaction rates dependent on the concentration of the catalyst and substrate . The kinetics of these reactions have been studied extensively, revealing that the rate constants for oxygen transfer can range from 0.1 to 3 L mol⁻¹ s⁻¹.
Table 1: Oxidation Rates of Sulfoxides Using MTO
Substrate Type | Reaction Rate (L mol⁻¹ s⁻¹) |
---|---|
Dialkyl sulfoxides | 0.1 - 3 |
Diaryl sulfoxides | Variable |
Epoxidation Reactions
MTO has gained prominence in the epoxidation of alkenes, where it acts as a catalyst in conjunction with various oxidizing agents such as hydrogen peroxide and sodium percarbonate. The use of Lewis bases like pyridine has been shown to enhance the efficiency of MTO-catalyzed reactions by stabilizing intermediates and increasing catalytic turnover .
Table 2: Epoxidation Reactions Catalyzed by MTO
Oxidizing Agent | Reaction Conditions | Yield (%) |
---|---|---|
Hydrogen Peroxide | Aqueous solution | High |
Urea-Hydrogen Peroxide | Mild conditions | Moderate |
Sodium Percarbonate | Room temperature | High |
Case Study 1: Atom-Transfer Reactions
In a study conducted by Espenson et al., MTO was utilized to catalyze atom-transfer reactions involving hydrogen peroxide. The mechanism involved the electrophilic activation of hydrogen peroxide, allowing for efficient oxygen transfer without radical intermediates. This method demonstrated a cleaner reaction pathway compared to traditional oxidizing agents .
Case Study 2: Oxyfunctionalization Reactions
MTO has also been applied in oxyfunctionalization reactions, where it selectively introduces functional groups into organic molecules. The compound's ability to facilitate these transformations while maintaining high selectivity and yield has made it a valuable tool in synthetic organic chemistry .
Mechanism of Action
The catalytic activity of methyltrioxorhenium(VII) primarily involves the activation of hydrogen peroxide (H₂O₂) through an electrophilic mechanism. The compound transfers a single oxygen atom to the substrate, facilitating the oxidation process without generating free-radical intermediates . This mechanism ensures high selectivity and efficiency in the catalytic reactions.
Comparison with Similar Compounds
Catalytic Efficiency and Selectivity
Rhenium-Based Catalysts
MTO is the most active rhenium-based oxidation catalyst. Comparative studies highlight its superiority over other Re(VII) compounds, such as NH₄ReO₄ and Al(ReO₄)₃, which are typically used in heterogeneous systems. These compounds require high temperatures (>100°C) for alkene epoxidation, whereas MTO operates efficiently at room temperature .
Non-Rhenium Catalysts
- Vanadium Catalysts : Vanadium oxo complexes (e.g., VO(O₂)⁺) are less effective in oxidizing sulfur-containing substrates. For instance, MTO achieves >90% sulfoxide yield from sulfides, whereas vanadium catalysts require harsher conditions (e.g., strong acids) and show lower chemoselectivity .
- Molybdenum/Tungsten Polyoxometalates: These catalysts are effective in H₂O₂-mediated oxidations but lack MTO’s regioselectivity. For example, MTO selectively oxidizes β-pinene to verbenol, while Mo-based systems produce complex mixtures .
Ligand Effects and Reaction Mechanisms
MTO’s activity is modulated by Lewis base ligands (e.g., pyridines, pyrazoles), which stabilize peroxo intermediates and enhance epoxidation rates . However, chiral ligands often require excess quantities (up to 100 mol%), limiting enantioselectivity in asymmetric epoxidation . In contrast, Schiff base-modified MTO complexes (e.g., CH₃ReO₃-salen adducts) exhibit shorter Re-O bonds and reduced catalytic activity, highlighting a trade-off between stability and efficiency .
Comparatively, organoruthenium polyoxometalates achieve higher enantioselectivity (>80% ee) in adamantane hydroxylation but require stoichiometric oxidants like 2,6-dichloropyridine N-oxide, increasing costs .
Environmental and Industrial Applicability
MTO operates under greener conditions than many alternatives. For instance:
- C–C Bond Cleavage : MTO/H₂O₂ cleaves lignin model compounds (e.g., β-O-4 linkages) with >98% conversion, outperforming Fe- or Mn-porphyrin catalysts that require toxic co-oxidants .
- Thiophene Oxidation : MTO oxidizes thiophene derivatives to sulfoxides with 85–95% yield, whereas Ti-silicates achieve <60% yield under similar conditions .
However, MTO’s high cost and rhenium scarcity limit large-scale adoption. Heterogenized Re₂O₇ on Al₂O₃ offers a cheaper alternative but with reduced TONs (~1,000) .
Stability and Deactivation Pathways
MTO is prone to deactivation via peroxide degradation, forming inactive perrhenate (ReO₄⁻) under prolonged H₂O₂ exposure . This contrasts with more robust catalysts like methylrhenium diimide complexes, which resist decomposition but show lower activity .
Biological Activity
Methyltrioxorhenium(VII) (MTO) is a rhenium-based compound that has garnered significant attention due to its catalytic properties and potential biological applications. This article explores the biological activity of MTO, focusing on its toxicity, catalytic mechanisms, and applications in various biochemical processes.
MTO is characterized by its ability to activate hydrogen peroxide (H2O2) through an electrophilic mechanism, facilitating the transfer of oxygen atoms to various substrates without generating free radicals. This property makes it an effective catalyst in oxidation reactions, particularly in olefin epoxidation and aldehyde olefination . The reaction mechanism typically involves the formation of peroxorhenium intermediates, which play a crucial role in the catalytic cycle .
Table 1: Key Properties of Methyltrioxorhenium(VII)
Property | Value |
---|---|
Molecular Formula | CH₃ReO₃ |
Molecular Weight | 214.2 g/mol |
Oxidation State | +7 |
Solubility | Soluble in polar solvents |
Toxicity | Moderate (dependent on concentration) |
Biological Activity and Toxicity
The biological activity of MTO has been assessed through various studies focusing on its cytotoxicity and genotoxicity. Notably, MTO has been evaluated for its inhibitory effects on acetylcholinesterase (AChE), an enzyme critical for neurotransmission. In vitro assays demonstrated that MTO exhibits significant inhibition of AChE, with half-maximal effective concentrations (EC50) indicating a potent interaction with this enzyme .
Case Study: Acetylcholinesterase Inhibition
In a study assessing the toxicity of MTO, isolated electric eel AChE was used to measure the compound's inhibitory effects. The results indicated that MTO's inhibition was dose-dependent, with significant activity observed at concentrations as low as 500 µM. The study also compared the effects of MTO with its degradation products, highlighting that while MTO is biologically active, its by-products exhibited lower toxicity levels .
Table 2: Inhibitory Concentrations of Methyltrioxorhenium(VII)
Compound | EC50 (µM) | Toxicity Level |
---|---|---|
Methyltrioxorhenium(VII) | 250 | Moderate |
MTO-TBP | 300 | Low |
NH₄ReO₄ | 1000 | Minimal |
Environmental Impact and Ecotoxicity
The ecotoxicological assessment of MTO has revealed that it poses moderate risks to aquatic organisms. Studies have shown that exposure to MTO can lead to significant toxicity in species such as Daphnia magna and Raphidocelis subcapitata, with observed effects including reduced viability and growth inhibition . The degradation products of MTO, particularly perrhenate, exhibit lower toxicity, suggesting that careful management of MTO usage is essential to minimize environmental risks.
Table 3: Ecotoxicological Effects of Methyltrioxorhenium(VII)
Organism | Concentration (µM) | Observed Effect |
---|---|---|
Daphnia magna | 500 | Reduced mobility |
Raphidocelis subcapitata | 200 | Growth inhibition |
Vibrio fischeri | 100 | Luminescence reduction |
Applications in Biocatalysis
MTO's unique properties have led to its application in biocatalytic processes. Its ability to facilitate oxidation reactions under mild conditions makes it suitable for various biochemical applications, including:
- Epoxidation of Olefins : MTO is widely used in organic synthesis for the selective epoxidation of alkenes.
- Dehydrogenation Reactions : It catalyzes dehydrogenation processes, contributing to the production of fine chemicals.
- Environmental Remediation : Due to its oxidative capabilities, MTO has potential applications in the degradation of environmental pollutants.
Q & A
Basic Research Questions
Q. What are the foundational synthetic methodologies for preparing Methyltrioxorhenium(VII), and how do experimental conditions influence yield and purity?
MTO is synthesized via the reaction of methylrhenium trioxide precursors with oxidizing agents like hydrogen peroxide. Key factors include:
- Temperature control : Elevated temperatures (>80°C) risk decomposition, while lower temperatures (<50°C) prolong reaction times .
- Solvent selection : Polar aprotic solvents (e.g., dichloromethane) minimize side reactions, whereas protic solvents can destabilize MTO .
- Purification : Sublimation under vacuum (40–60°C) yields high-purity MTO (>98%), critical for catalytic applications .
Q. How does MTO’s Lewis acidity compare to other transition metal catalysts, and what experimental techniques validate this property?
MTO’s Lewis acidity is quantified via spectrophotometric titration with N-donor ligands (e.g., pyridine derivatives) in non-polar solvents (CCl₄). Formation constants (K_f) and enthalpies (ΔH) derived from UV-Vis data correlate with Hammett σ constants, establishing a σ-acceptor strength scale. For example, K_f values for pyridine adducts range from 1.2 × 10³ to 5.6 × 10³ M⁻¹, confirming MTO’s moderate-to-strong Lewis acidity .
Q. What are the standard protocols for characterizing MTO’s structure and purity in solution?
- NMR spectroscopy : ¹H NMR (CDCl₃) shows a singlet at δ 2.75 ppm for the methyl group; ¹⁸⁷Re NMR exhibits a resonance near −1,100 ppm .
- IR spectroscopy : Strong Re=O stretches at 970–985 cm⁻¹ and 905–920 cm⁻¹ .
- Elemental analysis : Carbon content deviations >0.3% indicate impurities .
Advanced Research Questions
Q. How can the ECW model be applied to predict MTO’s reactivity in Lewis acid-base adduct formation?
The ECW model uses parameters E_A (electrophilicity) and C_A (nucleophilicity) to quantify MTO’s interactions. For example:
- E_A = 2.45 and C_A = 0.78 for MTO, derived from enthalpies of adduct formation with pyridine derivatives.
- Linear regression of log K_f vs. Hammett σ constants (ρ = 1.12) validates the model’s predictive power for ligand-specific reactivity .
Q. What mechanistic insights explain MTO’s dual role in olefin oxidation and metathesis reactions?
- Oxidation : MTO activates H₂O₂ to form peroxo intermediates (e.g., CH₃ReO(O₂)₂), which epoxidize olefins via a radical pathway. Turnover frequencies (TOFs) exceed 500 h⁻¹ for styrene epoxidation .
- Metathesis : MTO’s Re=O bonds facilitate [2+2] cycloaddition with alkenes, forming metallacyclobutane intermediates. Substrate steric effects (e.g., 1,2-disubstituted alkenes) reduce TOFs by 40–60% compared to terminal alkenes .
Q. How do contradictory data on MTO’s stability in aerobic vs. anaerobic conditions arise, and how can they be resolved experimentally?
Discrepancies stem from:
- Oxygen sensitivity : MTO decomposes under prolonged aerobic conditions (>24 hrs) to ReO₄⁻, detectable via ICP-MS. Anaerobic storage (N₂ glovebox) extends stability to >1 month .
- Ligand effects : Addition of 2,6-lutidine (10 mol%) suppresses decomposition by stabilizing the Re center .
- Resolution : Use TGA to monitor mass loss (decomposition onset: 120°C) and XRD to confirm crystallinity post-storage .
Q. What strategies optimize MTO’s catalytic efficiency in large-scale oxidation reactions while minimizing rhenium leaching?
- Co-catalysts : Additives like NH₄VO₃ (5 mol%) enhance TOFs by 30% and reduce Re leaching to <0.1 ppm via redox buffering .
- Solvent engineering : Biphasic systems (H₂O/CH₂Cl₂) recover >95% MTO through phase separation .
- Support immobilization : Grafting MTO onto mesoporous SiO₂ (pore size: 3–5 nm) increases recyclability (≥10 cycles) without activity loss .
Q. Methodological Guidance
Q. How should researchers design kinetic studies to differentiate between homogeneous and heterogeneous catalytic pathways in MTO-mediated reactions?
- Mercury poisoning test : Adding Hg(0) (10 equiv) suppresses heterogeneous pathways (e.g., nanoparticle formation). A >50% drop in TOF indicates dominant homogeneous catalysis .
- Dynamic light scattering (DLS) : Monitor particle size; aggregates >5 nm suggest heterogeneous mechanisms .
- Hot filtration test : Remove catalyst mid-reaction; continued conversion implies leaching or homogeneous activity .
Q. What statistical approaches are recommended for analyzing contradictory spectroscopic data in MTO-ligand adduct studies?
- Multivariate regression : Correlate UV-Vis absorbance (λ = 450–550 nm) with ligand σ parameters (R² > 0.95) .
- Error analysis : Use Student’s t-test to compare K_f values across solvents (e.g., CCl₄ vs. CH₃CN; p < 0.05 confirms solvent effects) .
Q. How can computational methods (DFT, MD) complement experimental studies of MTO’s reaction mechanisms?
- Transition state modeling : DFT (B3LYP/def2-TZVP) identifies key intermediates in epoxidation (e.g., peroxo vs. oxo pathways, ΔG‡ = 15–20 kcal/mol) .
- Solvent effects : MD simulations (AMBER) quantify H-bonding interactions in aqueous H₂O₂, explaining rate enhancements in polar media .
Q. Tables for Key Data
Property | Value | Method | Reference |
---|---|---|---|
Lewis acidity (E_A) | 2.45 | ECW model (ΔH titration) | |
¹H NMR (δ, ppm) | 2.75 (singlet) | CDCl₃, 400 MHz | |
Decomposition onset | 120°C | TGA | |
Epoxidation TOF (styrene) | 500 h⁻¹ | GC-MS |
Properties
IUPAC Name |
carbanide;trioxorhenium | |
---|---|---|
Source | PubChem | |
URL | https://pubchem.ncbi.nlm.nih.gov | |
Description | Data deposited in or computed by PubChem | |
InChI |
InChI=1S/CH3.3O.Re/h1H3;;;;/q-1;;;; | |
Source | PubChem | |
URL | https://pubchem.ncbi.nlm.nih.gov | |
Description | Data deposited in or computed by PubChem | |
InChI Key |
VZSXFJPZOCRDPW-UHFFFAOYSA-N | |
Source | PubChem | |
URL | https://pubchem.ncbi.nlm.nih.gov | |
Description | Data deposited in or computed by PubChem | |
Canonical SMILES |
[CH3-].O=[Re](=O)=O | |
Source | PubChem | |
URL | https://pubchem.ncbi.nlm.nih.gov | |
Description | Data deposited in or computed by PubChem | |
Molecular Formula |
CH3O3Re- | |
Source | PubChem | |
URL | https://pubchem.ncbi.nlm.nih.gov | |
Description | Data deposited in or computed by PubChem | |
DSSTOX Substance ID |
DTXSID40370077 | |
Record name | Methylrhenium trioxide | |
Source | EPA DSSTox | |
URL | https://comptox.epa.gov/dashboard/DTXSID40370077 | |
Description | DSSTox provides a high quality public chemistry resource for supporting improved predictive toxicology. | |
Molecular Weight |
249.24 g/mol | |
Source | PubChem | |
URL | https://pubchem.ncbi.nlm.nih.gov | |
Description | Data deposited in or computed by PubChem | |
CAS No. |
70197-13-6 | |
Record name | Methyltrioxorhenium | |
Source | ChemIDplus | |
URL | https://pubchem.ncbi.nlm.nih.gov/substance/?source=chemidplus&sourceid=0070197136 | |
Description | ChemIDplus is a free, web search system that provides access to the structure and nomenclature authority files used for the identification of chemical substances cited in National Library of Medicine (NLM) databases, including the TOXNET system. | |
Record name | Methylrhenium trioxide | |
Source | EPA DSSTox | |
URL | https://comptox.epa.gov/dashboard/DTXSID40370077 | |
Description | DSSTox provides a high quality public chemistry resource for supporting improved predictive toxicology. | |
Record name | Methylrhenium(VII) trioxide | |
Source | European Chemicals Agency (ECHA) | |
URL | https://echa.europa.eu/information-on-chemicals | |
Description | The European Chemicals Agency (ECHA) is an agency of the European Union which is the driving force among regulatory authorities in implementing the EU's groundbreaking chemicals legislation for the benefit of human health and the environment as well as for innovation and competitiveness. | |
Explanation | Use of the information, documents and data from the ECHA website is subject to the terms and conditions of this Legal Notice, and subject to other binding limitations provided for under applicable law, the information, documents and data made available on the ECHA website may be reproduced, distributed and/or used, totally or in part, for non-commercial purposes provided that ECHA is acknowledged as the source: "Source: European Chemicals Agency, http://echa.europa.eu/". Such acknowledgement must be included in each copy of the material. ECHA permits and encourages organisations and individuals to create links to the ECHA website under the following cumulative conditions: Links can only be made to webpages that provide a link to the Legal Notice page. | |
Record name | METHYLTRIOXORHENIUM | |
Source | FDA Global Substance Registration System (GSRS) | |
URL | https://gsrs.ncats.nih.gov/ginas/app/beta/substances/883D8RDD5Q | |
Description | The FDA Global Substance Registration System (GSRS) enables the efficient and accurate exchange of information on what substances are in regulated products. Instead of relying on names, which vary across regulatory domains, countries, and regions, the GSRS knowledge base makes it possible for substances to be defined by standardized, scientific descriptions. | |
Explanation | Unless otherwise noted, the contents of the FDA website (www.fda.gov), both text and graphics, are not copyrighted. They are in the public domain and may be republished, reprinted and otherwise used freely by anyone without the need to obtain permission from FDA. Credit to the U.S. Food and Drug Administration as the source is appreciated but not required. | |
Disclaimer and Information on In-Vitro Research Products
Please be aware that all articles and product information presented on BenchChem are intended solely for informational purposes. The products available for purchase on BenchChem are specifically designed for in-vitro studies, which are conducted outside of living organisms. In-vitro studies, derived from the Latin term "in glass," involve experiments performed in controlled laboratory settings using cells or tissues. It is important to note that these products are not categorized as medicines or drugs, and they have not received approval from the FDA for the prevention, treatment, or cure of any medical condition, ailment, or disease. We must emphasize that any form of bodily introduction of these products into humans or animals is strictly prohibited by law. It is essential to adhere to these guidelines to ensure compliance with legal and ethical standards in research and experimentation.