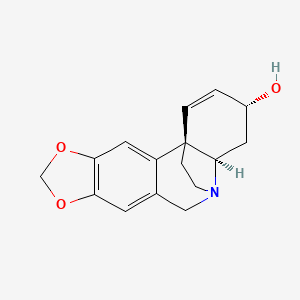
Crinine
- Click on QUICK INQUIRY to receive a quote from our team of experts.
- With the quality product at a COMPETITIVE price, you can focus more on your research.
Overview
Description
Crinine is a bioactive isoquinoline alkaloid isolated primarily from plants of the Amaryllidaceae family, notably the Crinum genus . Its core structure consists of a 2,3,4,4a-tetrahydro-1H,6H-5,10b-ethanophenanthridine skeleton (Figure 1), which is synthesized via Pictet-Spengler cyclization during its biosynthetic pathway . This compound exhibits diverse pharmacological activities, including antitumor, anticholinesterase, and analgesic properties. Notably, (±)-crinine derivatives have demonstrated significant analgesic activity in preclinical studies , while this compound-type alkaloids like crinsarnine and sarniensinol inhibit tumor cell proliferation .
Scientific Research Applications
Biological Activities of Crinine
This compound exhibits a remarkable range of biological effects, which have been documented through various studies:
- Antiviral Properties : this compound has shown promise in combating viral infections, although specific mechanisms remain to be fully elucidated .
- Antibacterial Effects : Research indicates that this compound possesses antibacterial activity, making it a candidate for developing new antimicrobial agents .
- Anti-cancer Potential : Studies have demonstrated that this compound can induce apoptosis in cancer cells, suggesting its role as a potential anti-cancer agent .
- Anti-malarial and Anti-plasmodial Activities : this compound has been evaluated for its effectiveness against malaria parasites, showing potential as a treatment option .
Synthesis of this compound
The synthesis of this compound-type alkaloids has been a focal point of research due to their complex structures and biological significance. Recent advancements in synthetic methodologies include:
- Enantioselective Synthesis : Bioinspired approaches utilizing iridium-catalyzed asymmetric hydrogenation have enabled the efficient synthesis of this compound-type alkaloids. This method allows for high yields and enantioselectivity .
- Synthetic Pathways : Various synthetic routes have been developed over the years, including the use of cyclohexenones and Claisen-type reactions to create key intermediates leading to this compound .
Clinical Applications
A notable clinical study compared the efficacy and tolerability of vaginal formulations containing this compound derivatives (e.g., Crinone 8% gel) for luteal support in in vitro fertilization (IVF) cycles. The findings indicated comparable pregnancy rates with improved tolerability for the this compound formulation .
Pharmacological Studies
A comprehensive review highlighted the pharmacological actions of this compound alongside other related alkaloids. It emphasized the need for further research to clarify the mechanisms underlying its biological activities and potential therapeutic uses .
Data Table: Summary of Biological Activities
Q & A
Basic Research Questions
Q. What experimental protocols are recommended for isolating crinine from plant sources while minimizing degradation of its alkaloid structure?
To isolate this compound, prioritize cold extraction (e.g., methanol or ethanol at 4°C) followed by liquid-liquid partitioning to preserve labile functional groups. Column chromatography (silica gel, gradient elution with chloroform:methanol) is standard, but validate purity via HPLC-DAD/MS . Note that plant matrix complexity may require iterative purification steps; document retention times and spectral data for reproducibility .
Q. How should researchers design preliminary pharmacological assays to evaluate this compound’s bioactivity without overinterpreting false positives?
Adopt a tiered approach:
In vitro cytotoxicity screening (e.g., MTT assay on cancer cell lines with IC50 calculations).
Selectivity testing against non-target cells (e.g., normal fibroblasts).
Mechanistic follow-up (e.g., apoptosis markers via flow cytometry).
Include positive controls (e.g., doxorubicin) and statistical validation (ANOVA, p<0.05) to distinguish noise from true activity .
Advanced Research Questions
Q. How can contradictory data on this compound’s neuroprotective vs. neurotoxic effects be reconciled in preclinical models?
Address contradictions by:
- Dose-response standardization : this compound’s biphasic effects (e.g., antioxidant at low doses, pro-oxidant at high doses) require precise dosing intervals .
- Model specificity : Test across multiple models (e.g., Aβ-induced vs. glutamate-induced neurotoxicity in primary neurons).
- Omics integration : Pair phenotypic data with transcriptomics/proteomics to identify pathway crosstalk . Publish raw datasets to enable meta-analyses .
Q. What computational and experimental strategies validate this compound’s structure-activity relationships (SAR) for analog synthesis?
Molecular docking : Screen this compound analogs against target proteins (e.g., acetylcholinesterase) using AutoDock Vina, prioritizing compounds with ΔG < -8 kcal/mol .
Stereochemical analysis : Use X-ray crystallography or NOESY NMR to confirm 3D configurations critical for binding .
In vitro validation : Compare synthetic analogs’ bioactivity to natural this compound, adjusting substituents (e.g., hydroxylation patterns) to optimize potency .
Q. How do researchers resolve discrepancies in this compound’s reported pharmacokinetic profiles across species?
- Interspecies metabolomics : Compare liver microsome assays (human vs. rodent) to identify CYP450-mediated metabolic variations .
- Bioavailability optimization : Use nanoformulations (e.g., liposomes) to enhance solubility and plasma half-life in poorly absorbing models .
- PBPK modeling : Integrate in vitro ADME data into physiologically based models to predict in vivo behavior .
Q. Methodological Guidelines from Evidence Synthesis
Comparison with Similar Compounds
Comparison with Structurally Related Alkaloids
Crinine belongs to the this compound-type subgroup of Amaryllidaceae alkaloids, which share a phenanthridine core but differ in substituents and stereochemistry. Below is a comparative analysis with key analogs:
Table 1: Structural Features of this compound and Related Alkaloids
Key Observations :
- This compound and haemanthamine are enantiomeric scaffolds differentiated by optical rotation (OR) and ORD calculations, eliminating the need for complex spectroscopic analysis .
- Lycorine and sanguinine lack the ethanophenanthridine core, resulting in distinct activity profiles (e.g., lycorine’s antiviral activity vs. This compound’s antitumor effects) .
Pharmacological Activity Comparison
Key Findings :
- Haemanthamine exhibits superior antitumor potency (IC50 = 0.5–5 µM) compared to this compound, likely due to methoxy substitution enhancing cell permeability .
- Sanguinine is the most potent AChE inhibitor in this group, making it a candidate for Alzheimer’s disease therapy .
Figures and Tables
- Figure 1 : Core structure of this compound (included as a chemical drawing).
- Tables 1–3 : As presented above.
Preparation Methods
Historical Foundations of Crinine Synthesis
Early Approaches: Wildman’s Pictet–Spengler Cyclization
The first synthesis of the crinane framework was reported by Wildman in 1956, employing a Pictet–Spengler cyclization as the pivotal step . Starting from α-arylated cyclohexanone (±)-14 , keto-ester (±)-15 was synthesized and converted into hydrazine hydrazide (±)-16 . Treatment with nitrous acid yielded cyclic imine (±)-17 , which underwent catalytic hydrogenation to produce octahydroindole (±)-12 . Subsequent Pictet–Spengler cyclization with formaldehyde generated the tetracyclic crinane framework (±)-1 (Scheme 2) . While groundbreaking, this route produced racemic material and required multiple functional group interconversions, limiting its practicality.
Muxfeldt’s Total Synthesis: Claisen Reaction and Allylic Oxidation
In 1966, Muxfeldt achieved the first total synthesis of (±)-crinine through a Claisen reaction to establish the quaternary carbon center . β-Keto-ester (±)-21 was subjected to Claisen condensation, yielding lactam (±)-23 after saponification. Reduction with lithium aluminium hydride afforded C3a-arylated hexahydroindole (±)-24 , which underwent Pictet–Spengler cyclization to tetracyclic intermediate (±)-25 . Allylic oxidation using SeO₂ introduced the C6 hydroxyl group, completing the synthesis of (±)-crinine [(±)-26 ] . This 14-step sequence demonstrated the utility of allylic oxidation for late-stage functionalization but suffered from moderate yields (45% combined yield for key steps).
Modern Catalytic Strategies
Iridium-Catalyzed Asymmetric Hydrogenation
A bioinspired enantioselective synthesis of this compound-type alkaloids was developed in 2017, utilizing iridium-catalyzed asymmetric hydrogenation of racemic oxocrinines . Racemic oxocrinines 1 were prepared in four steps via biomimetic oxidative coupling of O-methylnorbelladine. Using chiral iridium catalysts, hydrogenation resolved racemic 1 into enantiomerically enriched dihydrocrinines 2c , which were debenzylated with BCl₃ to yield natural crinines like (+)-siculine and (−)-8-O-demethylmaritidine . This method achieved gram-scale production with >90% enantiomeric excess (ee), offering a concise route to diverse this compound analogs (Scheme 2) .
Palladium-Catalyzed Intramolecular Alder-Ene Reaction
In 2011, a 13-step synthesis of racemic hamayne (a this compound analog) featured a Pd-catalyzed intramolecular Alder-ene (IMAE) reaction . Starting from gem-dibromocyclopropane 7 , sulfonamide (±)-12 underwent IMAE to form C3a-arylhexahydroindole (±)-16 . Pictet–Spengler cyclization with formaldehyde then yielded the crinane core, demonstrating the versatility of transition-metal catalysis for constructing complex polycycles . While the overall yield was modest (≈15%), this approach highlighted the potential of Pd catalysis in alkaloid synthesis.
Stereocontrolled and Biomimetic Approaches
Keck’s Carbonyl-Ene Reaction
Keck’s 1990s work utilized a thermally induced carbonyl-ene reaction to assemble the crinane framework . Racemic allylic carbonate (±)-58 underwent ene cyclization to form hydroxamic acid (±)-58 , which was converted into lactam (±)-59 . Reduction with LiAlH₄ yielded amine (±)-12 , and Pictet–Spengler cyclization with Eschenmoser’s salt provided crinane [(±)-1 ] in 95% yield . This method improved upon Wildman’s by enhancing cyclization efficiency but retained racemic outcomes.
Mori’s Asymmetric Synthesis via Carbonyl-Ene Reaction
Mori’s 2020 asymmetric synthesis of (+)-crinamine employed a chiral palladium catalyst to induce enantioselectivity in a carbonyl-ene reaction . Starting from allylic carbonate 60 , asymmetric cyclization generated hydroxamic acid 61 with 92% ee. Subsequent steps mirrored Keck’s protocol, achieving the first enantioselective synthesis of a this compound alkaloid. This method underscores the role of asymmetric catalysis in accessing natural enantiomers.
Comparative Analysis of Synthetic Routes
Method | Key Steps | Steps | Yield | Stereocontrol |
---|---|---|---|---|
Wildman (1956) | Pictet–Spengler cyclization | 8 | 30% | Racemic |
Muxfeldt (1966) | Claisen reaction, allylic oxidation | 14 | 12% | Racemic |
Iridium catalysis (2017) | Asymmetric hydrogenation | 6 | 65% | >90% ee |
Pd-Alder-ene (2011) | Intramolecular Alder-ene reaction | 13 | 15% | Racemic |
Mori (2020) | Asymmetric carbonyl-ene reaction | 10 | 28% | 92% ee |
Table 1: Comparative evaluation of this compound syntheses highlighting step economy and stereochemical outcomes .
Properties
Molecular Formula |
C16H17NO3 |
---|---|
Molecular Weight |
271.31 g/mol |
IUPAC Name |
(1S,13R,15R)-5,7-dioxa-12-azapentacyclo[10.5.2.01,13.02,10.04,8]nonadeca-2,4(8),9,16-tetraen-15-ol |
InChI |
InChI=1S/C16H17NO3/c18-11-1-2-16-3-4-17(15(16)6-11)8-10-5-13-14(7-12(10)16)20-9-19-13/h1-2,5,7,11,15,18H,3-4,6,8-9H2/t11-,15+,16+/m0/s1 |
InChI Key |
RPAORVSEYNOMBR-IUIKQTSFSA-N |
SMILES |
C1CN2CC3=CC4=C(C=C3C15C2CC(C=C5)O)OCO4 |
Isomeric SMILES |
C1CN2CC3=CC4=C(C=C3[C@]15[C@H]2C[C@H](C=C5)O)OCO4 |
Canonical SMILES |
C1CN2CC3=CC4=C(C=C3C15C2CC(C=C5)O)OCO4 |
Synonyms |
crinine |
Origin of Product |
United States |
Retrosynthesis Analysis
AI-Powered Synthesis Planning: Our tool employs the Template_relevance Pistachio, Template_relevance Bkms_metabolic, Template_relevance Pistachio_ringbreaker, Template_relevance Reaxys, Template_relevance Reaxys_biocatalysis model, leveraging a vast database of chemical reactions to predict feasible synthetic routes.
One-Step Synthesis Focus: Specifically designed for one-step synthesis, it provides concise and direct routes for your target compounds, streamlining the synthesis process.
Accurate Predictions: Utilizing the extensive PISTACHIO, BKMS_METABOLIC, PISTACHIO_RINGBREAKER, REAXYS, REAXYS_BIOCATALYSIS database, our tool offers high-accuracy predictions, reflecting the latest in chemical research and data.
Strategy Settings
Precursor scoring | Relevance Heuristic |
---|---|
Min. plausibility | 0.01 |
Model | Template_relevance |
Template Set | Pistachio/Bkms_metabolic/Pistachio_ringbreaker/Reaxys/Reaxys_biocatalysis |
Top-N result to add to graph | 6 |
Feasible Synthetic Routes
Disclaimer and Information on In-Vitro Research Products
Please be aware that all articles and product information presented on BenchChem are intended solely for informational purposes. The products available for purchase on BenchChem are specifically designed for in-vitro studies, which are conducted outside of living organisms. In-vitro studies, derived from the Latin term "in glass," involve experiments performed in controlled laboratory settings using cells or tissues. It is important to note that these products are not categorized as medicines or drugs, and they have not received approval from the FDA for the prevention, treatment, or cure of any medical condition, ailment, or disease. We must emphasize that any form of bodily introduction of these products into humans or animals is strictly prohibited by law. It is essential to adhere to these guidelines to ensure compliance with legal and ethical standards in research and experimentation.