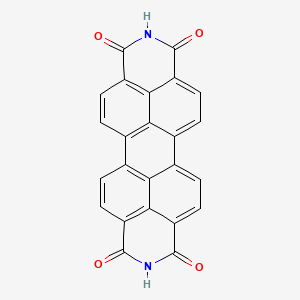
3,4,9,10-Perylenetetracarboxylic Diimide
Overview
Description
3,4,9,10-Perylenetetracarboxylic Diimide (PTCDI) is a planar aromatic compound derived from perylene tetracarboxylic dianhydride (PTCDA) via imidization. Its rigid π-conjugated structure enables strong intermolecular π-π stacking, making it a prominent n-type organic semiconductor . PTCDI exhibits high electron affinity (LUMO ~−4.0 eV), thermal stability (decomposition temperatures >300°C), and redox activity, which are exploited in organic electronics (e.g., field-effect transistors, solar cells) and energy storage (e.g., Li/Na/K-ion batteries) . However, its low solubility in organic solvents limits solution-based processing, prompting chemical modifications to enhance solubility and tailor properties .
Preparation Methods
Synthesis from Perylenetetracarboxylic Acid Dianhydride (PTCDA)
The reaction of perylenetetracarboxylic acid dianhydride (PTCDA) with ammonia or amines represents a foundational method for PTCDI synthesis. This route involves nucleophilic substitution at the anhydride groups, forming imide linkages. Early industrial processes employed concentrated sulfuric acid to hydrolyze PTCDI back to PTCDA, creating a cyclic dependency that limited cost-effectiveness . Modern adaptations avoid this by directly reacting PTCDA with ammonia under controlled conditions.
For example, PTCDA reacts with aqueous ammonia at 80–100°C to yield PTCDI with ≈85% purity. However, residual unreacted PTCDA and byproducts like monoimide derivatives necessitate post-synthetic purification via recrystallization from sulfuric acid . While straightforward, this method’s reliance on PTCDA—a compound historically derived from PTCDI—introduces economic inefficiencies, relegating it to small-scale laboratory use rather than bulk production.
Industrial Synthesis via Naphthalene-1,8-Dicarboxylic Acid Imide Fusion
To circumvent PTCDA dependency, industrial protocols favor naphthalene-1,8-dicarboxylic acid imide as a precursor. A patented process involves fusing this imide with potassium hydroxide (KOH) and sodium acetate (NaOAc) at 190–220°C under inert conditions . The reaction proceeds via aldol condensation, forming the leuco compound of PTCDI, which is subsequently oxidized to the final product.
Key Reaction Parameters:
Parameter | Optimal Range | Impact on Yield/Purity |
---|---|---|
Temperature | 190–220°C | Higher temps improve cyclization but risk decomposition |
KOH:NaOAc molar ratio | 1:1.5–2.0 | Excess NaOAc enhances solubility of intermediates |
Oxidation method | Aeration or peroxodisulfates | Aeration minimizes byproducts vs. chemical oxidants |
Post-reaction, the melt is diluted with water, and the leuco compound is oxidized via aeration or peroxodisulfates. This method achieves 90–95% purity and yields exceeding 85%, outperforming earlier approaches that struggled with impurities . The use of anhydrous KOH (10–15% water content) and sodium acetate prevents unwanted hydrolysis, ensuring consistent product quality.
Imidazole-Mediated Condensation for Functionalized Derivatives
Recent advances leverage imidazole as a high-boiling solvent (130°C) and catalyst for PTCDI functionalization. For instance, reacting PTCDA with amino-polyethylene glycol (NH₂-PEG-COOH) in imidazole yields water-soluble PTCDI derivatives in 70% yield . The solvent’s dual role as a base and reaction medium facilitates imide bond formation without requiring metal catalysts.
Case Study: Synthesis of PDI–COOH
-
Reactants : PTCDA (254.89 mmol), NH₂-PEG-10-COOH (764.68 mmol), imidazole (36 mmol).
-
Conditions : 130°C, 5 hours under reflux.
-
Workup : Extraction with chloroform, acid washing, and vacuum drying.
-
Yield : 70% (red oily liquid).
This method’s modularity allows substitution with diverse amines (e.g., aliphatic, aromatic) to tailor PTCDI’s electronic properties. For example, pentafluorophenol esterification of PDI–COOH introduces electrophilic sites for further conjugation . However, scalability is limited by imidazole’s viscosity and the need for rigorous solvent recovery.
Alkali Fusion with Metal Acetate Additives
Modifying the industrial fusion process with metal acetates (e.g., zinc acetate) enhances reaction rates and product homogeneity. European Patent Application No. 0,054,806 details a protocol using KOH, NaOH, and NaOAc at 190–220°C, achieving 93.4% yield . However, independent validation revealed only 81% purity, prompting refinements such as:
-
Extended reaction times : 6–8 hours at 220°C to ensure complete cyclization.
-
Gradual cooling : Prevents premature crystallization of intermediates.
-
Acid washing : Removes residual alkali metals, yielding pigment-grade PTCDI .
Electrochemical Applications and Purity Requirements
PTCDI’s emergence as a sodium-ion battery cathode material necessitates ultrahigh purity (>99%) to ensure electrochemical stability . Traditional methods often fall short due to trace metal contaminants (e.g., K⁺, Na⁺), necessitating additional steps:
Chemical Reactions Analysis
Types of Reactions
3,4,9,10-Perylenetetracarboxylic Diimide undergoes various chemical reactions, including:
Oxidation: The compound can be oxidized to form perylene-3,4,9,10-tetracarboxylic dianhydride.
Reduction: Reduction reactions can convert the diimide to its corresponding dihydro derivative.
Substitution: The compound can undergo substitution reactions, particularly at the imide nitrogen atoms, to form various derivatives.
Common Reagents and Conditions
Oxidation: Common oxidizing agents include potassium permanganate and chromium trioxide.
Reduction: Reducing agents such as sodium borohydride and lithium aluminum hydride are often used.
Substitution: Substitution reactions typically involve nucleophiles such as amines or alcohols under basic conditions.
Major Products
The major products formed from these reactions include perylene-3,4,9,10-tetracarboxylic dianhydride, dihydro derivatives, and various substituted imides .
Scientific Research Applications
Organic Electronics
1.1. Electron Transport Materials
PTCDI derivatives are extensively used as electron transport materials in organic solar cells and organic light-emitting diodes (OLEDs). Their high electron mobility and stability make them suitable for these applications. For instance, tetrahydroxy-perylene bismide has shown effectiveness as an electron transport material in non-fullerene organic solar cells . Additionally, modifications at the bay positions of PTCDI have been shown to enhance its electron-transporting capabilities by altering the HOMO and LUMO energy levels, thus optimizing device performance .
1.2. Composite Electrodes
Recent studies have demonstrated that PTCDI can be utilized in composite electrodes for energy storage devices. Its incorporation into electrodes has resulted in improved charge-discharge rates and overall device efficiency. For example, H2PTCDI films enable remarkably fast charging/discharging rates due to their diffusion-less nature . This property is particularly beneficial for applications in supercapacitors and batteries.
Photonics
2.1. Light Harvesting Systems
PTCDI has been investigated for its role in mimicking natural light-harvesting systems through the formation of J-type aggregates. These aggregates exhibit unique photophysical properties, such as high fluorescence quantum yields and stability under thermal and photonic stress . The ability to tune the optical properties through structural modifications makes PTCDI an attractive candidate for developing advanced photonic devices.
2.2. Luminescence Sensors
Due to its strong luminescent properties, PTCDI is also studied for use in luminescence sensors. Its ability to act as an electron acceptor enhances its functionality in sensor applications, allowing for the detection of various analytes through fluorescence quenching mechanisms . The design of PTCDI derivatives with specific functional groups can further optimize their sensitivity and selectivity.
Nanotechnology
3.1. Colloidal Stability of Nanoparticles
PTCDI plays a significant role in enhancing the colloidal stability of gold nanoparticles when used as a coating agent . The structural constraints provided by PTCDI ligands improve the stability and functionality of these nanoparticles in various applications, including drug delivery and imaging.
3.2. Nonlinear Optical Applications
Research has indicated that PTCDI derivatives can be applied in nonlinear optical devices due to their unique electronic properties . Their ability to undergo two-photon absorption makes them suitable for applications in optical switching and signal processing.
Summary Table of Applications
Application Area | Specific Use Case | Key Properties |
---|---|---|
Organic Electronics | Electron transport materials | High electron mobility |
Composite electrodes | Fast charge/discharge rates | |
Photonics | Light harvesting systems | High fluorescence quantum yields |
Luminescence sensors | Enhanced sensitivity | |
Nanotechnology | Colloidal stability of nanoparticles | Improved stability |
Nonlinear optical applications | Two-photon absorption capability |
Mechanism of Action
The mechanism by which 3,4,9,10-Perylenetetracarboxylic Diimide exerts its effects is primarily through its interaction with light and electronic properties. The planar structure allows for efficient π-π stacking interactions, which are crucial for its function as a light-harvesting material and in optoelectronic applications . Additionally, the compound can form stable complexes with various metal ions, enhancing its utility in biological and chemical sensing .
Comparison with Similar Compounds
Structural and Functional Modifications
Substituent Effects on Solubility and Morphology
PTCDI derivatives are modified by substituting the imide nitrogen with alkyl, aryl, or heterocyclic groups. Key examples include:
- Alkyl Chains (DD-PTCDI/ND-PTCDI): Long alkyl chains (e.g., dodecyl) disrupt π-π interactions, enhancing solubility in organic solvents. DD-PTCDI forms nanobelts with strong intermolecular stacking, whereas ND-PTCDI forms nanoparticles due to steric hindrance .
- Heterocyclic Groups (DMP/DZP) : Pyrimidine or pyrazine substituents lower LUMO levels (−4.2 eV vs. −4.0 eV for PTCDI), improving electron transport and thermal stability (decomposition >350°C) .
Comparison in Battery Systems
- PTCDI vs. PTCDA : PTCDI’s imide groups enable reversible six-electron redox reactions, outperforming PTCDA (dianhydride) in K-ion batteries due to faster K⁺ diffusion (~10⁻⁸ cm²/s) and lower charge-transfer resistance (93 Ω) .
- Derivative-Specific Performance : Methyl/phenyl-substituted PTCDI derivatives (Me₂PTCDI/Ph₂PTCDI) show reduced capacity but enhanced cycle life in Li-ion systems, attributed to stabilized redox intermediates .
Optical and Electronic Properties
Substituent Impact on Optoelectronics
- Alkyl vs. Heterocyclic Substituents : PTCDI-C8 (octyl chains) exhibits red-shifted absorption/emission and higher charge mobility (0.4–0.6 cm²/V·s) due to ordered crystalline wires . Heterocyclic derivatives (DMP) achieve even higher mobility (0.8 cm²/V·s) and are stable under ambient conditions .
Thermal and Environmental Stability
- Thermal Stability : PTCDI derivatives with aromatic substituents (e.g., DMP) decompose at 350–400°C, outperforming alkylated derivatives (DD-PTCDI: ~300°C) .
- Oxidation Resistance : Nitrogen-containing heterocycles (pyrimidine) in DMP reduce oxidation susceptibility, critical for air-stable electronics .
Biological Activity
3,4,9,10-Perylenetetracarboxylic diimide (PTCDI) is a compound that has garnered significant attention in various fields, particularly in organic electronics and sensor technology. This article provides a comprehensive overview of the biological activity associated with PTCDI, including its applications as a sensor for environmental pollutants, its photophysical properties, and its potential therapeutic roles.
Overview of this compound
PTCDI is a derivative of perylene and is characterized by its strong electron-accepting properties and excellent thermal and chemical stability. Its structure allows for extensive functionalization, which can modify its electronic properties and enhance its applicability in various domains.
Biological Applications
1. Environmental Sensors
PTCDI and its derivatives have been extensively studied for their application in the development of fluorescent sensors. These sensors can detect heavy metal ions such as Cu²⁺ and Al³⁺ with high sensitivity. For instance, a study demonstrated that PDI-4 could effectively sense Cu²⁺ ions in aqueous solutions through fluorescence quenching mechanisms facilitated by micellar systems formed with cationic surfactants like cetyltrimethylammonium bromide (CTAB) .
2. Photophysical Properties
The photophysical properties of PTCDI are crucial for its application in sensors and organic photovoltaics. The compound exhibits strong visible-light absorption and unique fluorescence characteristics. DFT studies have shown that the functionalization at specific positions alters the HOMO-LUMO gap, impacting the absorption wavelengths and fluorescence efficiency .
3. Antioxidant Activity
Recent research indicates that PTCDI derivatives possess antioxidant properties. These compounds can scavenge free radicals effectively, suggesting potential applications in therapeutic contexts where oxidative stress is a concern. The mechanisms involve electron transfer processes that stabilize radical species .
Case Studies
Study 1: Fluorescent Sensor Development
In a notable study, PDI-7 was modified with di(2-(salicylideneamino))ethylamine (DSEA) to enhance its sensitivity towards Al³⁺ ions. The sensor exhibited a limit of detection (LOD) of 0.33 µM in acetonitrile solutions, showcasing the ability of PTCDI derivatives to form stable complexes with metal ions, leading to increased fluorescence intensity .
Study 2: Photovoltaic Applications
PTCDI has been evaluated as an electron transport material in organic solar cells. Research indicates that the incorporation of PTCDI into device architectures improves charge mobility and overall efficiency due to its favorable energy levels and electron affinity . This application highlights the compound's versatility beyond sensing.
Data Summary
The following table summarizes key findings related to the biological activity of PTCDI:
Q & A
Q. What are the standard synthetic routes for PTCDI and its derivatives, and how are they characterized?
Basic Research Focus
PTCDI is typically synthesized via condensation of 3,4,9,10-perylenetetracarboxylic dianhydride (PTCDA) with primary amines under inert conditions. Derivatives are created by varying substituents (alkyl, aryl, or functional groups) at the imide positions. For example, N,N′-bis(4-methoxyphenyl)-PTCDI is synthesized using 4-methoxybenzylamine .
Characterization Methods :
- NMR Spectroscopy : Confirms functionalization and purity .
- XRD : Analyzes crystallinity and π-π stacking .
- UV-Vis/PL Spectroscopy : Evaluates optical properties (e.g., absorption/emission shifts due to aggregation) .
- DSC/TGA : Assesses thermal stability and phase transitions .
Q. How do side-chain modifications influence the self-assembly morphology of PTCDI derivatives?
Advanced Research Focus
Side chains critically modulate intermolecular interactions and aggregation behavior:
Methodological Insights :
- SEM/TEM/AFM : Visualize nanostructure dimensions and topology .
- Fluorescence Microscopy : Correlates morphology with emission properties (e.g., red-shifted emission in DD-PTCDI due to closer π-π stacking) .
Q. What methodologies are used to assess PTCDI-based materials in energy storage applications?
Advanced Research Focus
PTCDI derivatives show promise in aqueous ammonium-ion batteries (AAIBs) due to redox-active imide groups:
- Cyclic Voltammetry (CV) : Quantifies redox potentials and reversibility .
- Galvanostatic Cycling : Measures capacity retention (e.g., PTCDI-urea polymer retains 80 mAh g⁻¹ over 500 cycles) .
- In Situ XRD/Raman : Tracks structural changes during ion insertion/extraction .
Key Challenges : Optimizing electrolyte compatibility and minimizing dissolution in aqueous systems .
Q. How can researchers resolve discrepancies in reported electronic properties of PTCDI derivatives?
Data Contradiction Analysis
Variations in LUMO levels (e.g., −4.1 eV for DMP vs. −3.7 eV for DPP ) arise from:
- Substituent Effects : Electron-withdrawing/donating groups alter orbital energies.
- Measurement Techniques : Electrochemical vs. computational (DFT) methods may differ.
Mitigation Strategies : - Standardize characterization protocols (e.g., CV under identical conditions).
- Cross-validate with DFT simulations to align experimental and theoretical values .
Q. What spectroscopic techniques are essential for analyzing PTCDI's optical properties?
Basic Research Focus
- UV-Vis Absorption : Identifies aggregation states (e.g., J-aggregates show bathochromic shifts) .
- Photoluminescence (PL) : Quantifies quantum yield and excimer formation (e.g., DD-PTCDI exhibits 3× lower quantum yield than ND-PTCDI) .
- Time-Resolved Spectroscopy : Probes exciton dynamics in films or solutions .
Q. How can PTCDI's charge transfer interactions with materials like C60 be optimized for organic electronics?
Advanced Research Focus
- Energy-Level Alignment : PTCDI derivatives with LUMO levels lower than C60 (e.g., DMP at −4.1 eV vs. C60 at −4.5 eV ) enable efficient electron transfer.
- Blend Morphology : Use solvent annealing or additives to control phase separation in bulk heterojunctions .
- Transient Absorption Spectroscopy : Maps charge separation efficiency in donor-acceptor systems .
Q. What are the key considerations in designing PTCDI derivatives for photovoltaic applications?
Basic Research Focus
Properties
IUPAC Name |
7,18-diazaheptacyclo[14.6.2.22,5.03,12.04,9.013,23.020,24]hexacosa-1(23),2,4,9,11,13,15,20(24),21,25-decaene-6,8,17,19-tetrone | |
---|---|---|
Source | PubChem | |
URL | https://pubchem.ncbi.nlm.nih.gov | |
Description | Data deposited in or computed by PubChem | |
InChI |
InChI=1S/C24H10N2O4/c27-21-13-5-1-9-10-2-6-15-20-16(24(30)26-23(15)29)8-4-12(18(10)20)11-3-7-14(22(28)25-21)19(13)17(9)11/h1-8H,(H,25,27,28)(H,26,29,30) | |
Source | PubChem | |
URL | https://pubchem.ncbi.nlm.nih.gov | |
Description | Data deposited in or computed by PubChem | |
InChI Key |
KJOLVZJFMDVPGB-UHFFFAOYSA-N | |
Source | PubChem | |
URL | https://pubchem.ncbi.nlm.nih.gov | |
Description | Data deposited in or computed by PubChem | |
Canonical SMILES |
C1=CC2=C3C(=CC=C4C3=C1C5=C6C4=CC=C7C6=C(C=C5)C(=O)NC7=O)C(=O)NC2=O | |
Source | PubChem | |
URL | https://pubchem.ncbi.nlm.nih.gov | |
Description | Data deposited in or computed by PubChem | |
Molecular Formula |
C24H10N2O4 | |
Source | PubChem | |
URL | https://pubchem.ncbi.nlm.nih.gov | |
Description | Data deposited in or computed by PubChem | |
DSSTOX Substance ID |
DTXSID9052555 | |
Record name | Pigment Violet 29 | |
Source | EPA DSSTox | |
URL | https://comptox.epa.gov/dashboard/DTXSID9052555 | |
Description | DSSTox provides a high quality public chemistry resource for supporting improved predictive toxicology. | |
Molecular Weight |
390.3 g/mol | |
Source | PubChem | |
URL | https://pubchem.ncbi.nlm.nih.gov | |
Description | Data deposited in or computed by PubChem | |
Physical Description |
Liquid, Other Solid | |
Record name | Anthra[2,1,9-def:6,5,10-d'e'f']diisoquinoline-1,3,8,10(2H,9H)-tetrone | |
Source | EPA Chemicals under the TSCA | |
URL | https://www.epa.gov/chemicals-under-tsca | |
Description | EPA Chemicals under the Toxic Substances Control Act (TSCA) collection contains information on chemicals and their regulations under TSCA, including non-confidential content from the TSCA Chemical Substance Inventory and Chemical Data Reporting. | |
CAS No. |
81-33-4, 12236-71-4 | |
Record name | Pigment Violet 29 | |
Source | CAS Common Chemistry | |
URL | https://commonchemistry.cas.org/detail?cas_rn=81-33-4 | |
Description | CAS Common Chemistry is an open community resource for accessing chemical information. Nearly 500,000 chemical substances from CAS REGISTRY cover areas of community interest, including common and frequently regulated chemicals, and those relevant to high school and undergraduate chemistry classes. This chemical information, curated by our expert scientists, is provided in alignment with our mission as a division of the American Chemical Society. | |
Explanation | The data from CAS Common Chemistry is provided under a CC-BY-NC 4.0 license, unless otherwise stated. | |
Record name | Perylimid | |
Source | ChemIDplus | |
URL | https://pubchem.ncbi.nlm.nih.gov/substance/?source=chemidplus&sourceid=0000081334 | |
Description | ChemIDplus is a free, web search system that provides access to the structure and nomenclature authority files used for the identification of chemical substances cited in National Library of Medicine (NLM) databases, including the TOXNET system. | |
Record name | Perylimid | |
Source | DTP/NCI | |
URL | https://dtp.cancer.gov/dtpstandard/servlet/dwindex?searchtype=NSC&outputformat=html&searchlist=16842 | |
Description | The NCI Development Therapeutics Program (DTP) provides services and resources to the academic and private-sector research communities worldwide to facilitate the discovery and development of new cancer therapeutic agents. | |
Explanation | Unless otherwise indicated, all text within NCI products is free of copyright and may be reused without our permission. Credit the National Cancer Institute as the source. | |
Record name | Anthra[2,1,9-def:6,5,10-d'e'f']diisoquinoline-1,3,8,10(2H,9H)-tetrone | |
Source | EPA Chemicals under the TSCA | |
URL | https://www.epa.gov/chemicals-under-tsca | |
Description | EPA Chemicals under the Toxic Substances Control Act (TSCA) collection contains information on chemicals and their regulations under TSCA, including non-confidential content from the TSCA Chemical Substance Inventory and Chemical Data Reporting. | |
Record name | Pigment Violet 29 | |
Source | EPA DSSTox | |
URL | https://comptox.epa.gov/dashboard/DTXSID9052555 | |
Description | DSSTox provides a high quality public chemistry resource for supporting improved predictive toxicology. | |
Record name | Perylene-3,4:9,10-tetracarboxydiimide | |
Source | European Chemicals Agency (ECHA) | |
URL | https://echa.europa.eu/substance-information/-/substanceinfo/100.001.223 | |
Description | The European Chemicals Agency (ECHA) is an agency of the European Union which is the driving force among regulatory authorities in implementing the EU's groundbreaking chemicals legislation for the benefit of human health and the environment as well as for innovation and competitiveness. | |
Explanation | Use of the information, documents and data from the ECHA website is subject to the terms and conditions of this Legal Notice, and subject to other binding limitations provided for under applicable law, the information, documents and data made available on the ECHA website may be reproduced, distributed and/or used, totally or in part, for non-commercial purposes provided that ECHA is acknowledged as the source: "Source: European Chemicals Agency, http://echa.europa.eu/". Such acknowledgement must be included in each copy of the material. ECHA permits and encourages organisations and individuals to create links to the ECHA website under the following cumulative conditions: Links can only be made to webpages that provide a link to the Legal Notice page. | |
Record name | Anthra[2,1,9-def:6,5,10-d'e'f']diisoquinoline-1,3,8,10(2H,9H)-tetrone | |
Source | European Chemicals Agency (ECHA) | |
URL | https://echa.europa.eu/information-on-chemicals | |
Description | The European Chemicals Agency (ECHA) is an agency of the European Union which is the driving force among regulatory authorities in implementing the EU's groundbreaking chemicals legislation for the benefit of human health and the environment as well as for innovation and competitiveness. | |
Explanation | Use of the information, documents and data from the ECHA website is subject to the terms and conditions of this Legal Notice, and subject to other binding limitations provided for under applicable law, the information, documents and data made available on the ECHA website may be reproduced, distributed and/or used, totally or in part, for non-commercial purposes provided that ECHA is acknowledged as the source: "Source: European Chemicals Agency, http://echa.europa.eu/". Such acknowledgement must be included in each copy of the material. ECHA permits and encourages organisations and individuals to create links to the ECHA website under the following cumulative conditions: Links can only be made to webpages that provide a link to the Legal Notice page. | |
Record name | 3,4,9,10-PERYLENETETRACARBOXYLIC DIIMIDE | |
Source | FDA Global Substance Registration System (GSRS) | |
URL | https://gsrs.ncats.nih.gov/ginas/app/beta/substances/63NLI8842L | |
Description | The FDA Global Substance Registration System (GSRS) enables the efficient and accurate exchange of information on what substances are in regulated products. Instead of relying on names, which vary across regulatory domains, countries, and regions, the GSRS knowledge base makes it possible for substances to be defined by standardized, scientific descriptions. | |
Explanation | Unless otherwise noted, the contents of the FDA website (www.fda.gov), both text and graphics, are not copyrighted. They are in the public domain and may be republished, reprinted and otherwise used freely by anyone without the need to obtain permission from FDA. Credit to the U.S. Food and Drug Administration as the source is appreciated but not required. | |
Retrosynthesis Analysis
AI-Powered Synthesis Planning: Our tool employs the Template_relevance Pistachio, Template_relevance Bkms_metabolic, Template_relevance Pistachio_ringbreaker, Template_relevance Reaxys, Template_relevance Reaxys_biocatalysis model, leveraging a vast database of chemical reactions to predict feasible synthetic routes.
One-Step Synthesis Focus: Specifically designed for one-step synthesis, it provides concise and direct routes for your target compounds, streamlining the synthesis process.
Accurate Predictions: Utilizing the extensive PISTACHIO, BKMS_METABOLIC, PISTACHIO_RINGBREAKER, REAXYS, REAXYS_BIOCATALYSIS database, our tool offers high-accuracy predictions, reflecting the latest in chemical research and data.
Strategy Settings
Precursor scoring | Relevance Heuristic |
---|---|
Min. plausibility | 0.01 |
Model | Template_relevance |
Template Set | Pistachio/Bkms_metabolic/Pistachio_ringbreaker/Reaxys/Reaxys_biocatalysis |
Top-N result to add to graph | 6 |
Feasible Synthetic Routes
Disclaimer and Information on In-Vitro Research Products
Please be aware that all articles and product information presented on BenchChem are intended solely for informational purposes. The products available for purchase on BenchChem are specifically designed for in-vitro studies, which are conducted outside of living organisms. In-vitro studies, derived from the Latin term "in glass," involve experiments performed in controlled laboratory settings using cells or tissues. It is important to note that these products are not categorized as medicines or drugs, and they have not received approval from the FDA for the prevention, treatment, or cure of any medical condition, ailment, or disease. We must emphasize that any form of bodily introduction of these products into humans or animals is strictly prohibited by law. It is essential to adhere to these guidelines to ensure compliance with legal and ethical standards in research and experimentation.