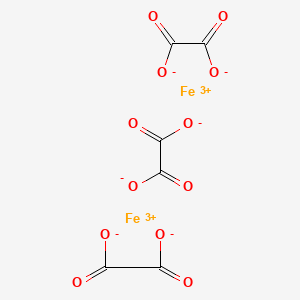
Iron oxalate
Overview
Description
Iron oxalate, primarily existing as iron(II) oxalate dihydrate (FeC₂O₄·2H₂O) and its anhydrous polymorphs (α-FeC₂O₄ and β-FeC₂O₄), is a coordination compound with significant industrial and scientific relevance. Structurally, it consists of Fe²⁺ cations bridged by oxalate (C₂O₄²⁻) ligands, forming chains stabilized by hydrogen bonds in the hydrated form . Recent interest in this compound stems from its applications in lithium-ion batteries due to its reversible lithium-ion intercalation capacity and environmental sustainability . Additionally, its thermal decomposition pathways yield iron oxides (e.g., Fe₃O₄, α-Fe₂O₃), making it a precursor for magnetic and catalytic materials .
Preparation Methods
Conventional Synthesis Methods
Precipitation from Ferrous Salts and Oxalic Acid
The most straightforward method involves reacting ferrous salts (e.g., Fe(NH₄)₂(SO₄)₂) with oxalic acid (H₂C₂O₄) under acidic conditions:
2\text{C}2\text{O}4 + 2\text{H}2\text{O} \rightarrow \text{FeC}2\text{O}4 + 2\text{H}_3\text{O}^+
In laboratory settings, this yields pale yellow FeC₂O₄ precipitates, which are washed with demineralized water to remove sulfate ions . Key parameters include:
-
Temperature : Heating to 40–60°C accelerates crystallization .
-
Acid concentration : Excess H₂C₂O₄ improves yield but complicates purification .
Table 1: Typical Yields from Ferrous Sulfate and Oxalic Acid Reactions
Ferrous Salt | Oxalic Acid (M) | Yield (%) | Purity (%) |
---|---|---|---|
Fe(NH₄)₂(SO₄)₂·6H₂O | 1.0 | 78 | 95 |
FeSO₄·7H₂O | 0.5 | 65 | 88 |
Oxidation of Iron(II) Oxalate to Iron(III) Oxalate
Ferrous oxalate is oxidized to Fe₂(C₂O₄)₃ using hydrogen peroxide (H₂O₂) in the presence of potassium oxalate:
2\text{O}4 + \text{H}2\text{O}2 + 2\text{H}3\text{O}^+ + \text{C}2\text{O}4^{2-} \rightarrow \text{Fe}2(\text{C}2\text{O}4)3 + 4\text{H}2\text{O}
This step requires precise pH control (3.5–4.0) and temperatures below 60°C to prevent peroxide decomposition . The resulting Fe₂(C₂O₄)₃ is sparingly soluble, necessitating filtration and ethanol washes for purification.
Sustainable and Waste-Valorization Approaches
Red Mud as a Feedstock
Red mud (RM), a bauxite processing waste rich in iron oxides, has been successfully converted to high-purity Fe₂(C₂O₄)₃ via acid leaching:
-
Leaching : RM is treated with 2M H₂C₂O₄ at 80°C for 4 hours, extracting 92% of Fe³⁺ .
-
Crystallization : The filtrate is cooled to 25°C, yielding 98% pure Fe₂(C₂O₄)₃·5H₂O crystals.
This method reduces reliance on virgin iron ores and aligns with circular economy principles.
Iron Ore Dissolution Using Oxalic Acid
A novel three-step process converts hematite (Fe₂O₃) to metallic iron via oxalate intermediates :
-
Dissolution : Fe₂O₃ reacts with H₂C₂O₄ to form Fe³⁺ oxalate complexes.
-
Photoreduction : UV light reduces Fe³⁺ to Fe²⁺, precipitating FeC₂O₄·2H₂O.
-
Pyrolysis : FeC₂O₄ decomposes at 400°C to yield Fe⁰ (96% purity).
Table 2: Performance of Different Iron Ores in Oxalic Acid Processes
Ore Type | Dissolution Efficiency (%) | Iron Purity (%) |
---|---|---|
Hematite | 89 | 96 |
Magnetite | 78 | 88 |
Goethite | 65 | 82 |
Specialized Laboratory Protocols
Potassium Trioxalatoferrate(III) Synthesis
A coordination complex, K₃[Fe(C₂O₄)₃], is prepared via:
-
Precipitation of FeC₂O₄ from Fe(NH₄)₂(SO₄)₂ and H₂C₂O₄.
-
Oxidation with H₂O₂ in the presence of K₂C₂O₄.
-
Complexation with additional H₂C₂O₄ to form the trioxalatoferrate anion .
The final product is isolated by adding ethanol, which reduces solubility, and is characterized by UV-Vis spectroscopy (λₘₐₓ = 510 nm) .
Ferric Oxalate Solution Preparation for Technical Applications
For photochemical uses, 27% w/w Fe₂(C₂O₄)₃ solutions are prepared by dissolving 27 g of crystals in 120 mL of water at 50–60°C . Post-dissolution, evaporation adjusts concentration, while filtration removes undissolved solids. Solution color indicates purity:
-
Golden amber : No free H₂C₂O₄ (ideal for platinum/palladium printing).
-
Yellow-green : Excess acid, requiring silver nitrate titration for quantification .
Analytical Techniques for this compound Characterization
-
Colorimetry : Beer’s Law plots (absorbance vs. concentration) validate Fe²⁺/Fe³⁺ ratios .
-
Titrimetry : Redox titrations with KMnO₄ determine oxalate content (RSD < 2%) .
-
Mass Spectrometry : Detects unusual oxidation states (e.g., Fe⁺ in [Fe(H₂O)₂]⁺) formed during reduction .
Challenges and Considerations in Preparation
-
Impurity Effects : Alkaline earth metals (Ca²⁺, Mg²⁺) co-precipitate with oxalates, reducing iron purity .
-
Stoichiometric Control : Excess H₂C₂O₄ stabilizes Fe³⁺ but complicates downstream applications like Kallitype printing .
-
Scalability : Batch processes dominate due to slow dissolution kinetics (e.g., 4 hours for RM leaching ).
Chemical Reactions Analysis
Types of Reactions: Iron oxalate undergoes various chemical reactions, including:
-
Oxidation: Iron(II) oxalate can be oxidized to iron(III) oxalate using hydrogen peroxide and potassium oxalate. [ 2 \text{FeC}_2\text{O}_4 + \text{H}_2\text{O}_2 + 2 \text{H}_3\text{O}^+ + \text{C}_2\text{O}_4^{2-} \rightarrow \text{Fe}_2(\text{C}_2\text{O}_4)_3 + 4 \text{H}_2\text{O} ]
-
Thermal Decomposition: When heated, this compound decomposes to form iron oxides, carbon dioxide, carbon monoxide, and water. [ \text{FeC}_2\text{O}_4 \rightarrow \text{FeO} + \text{CO}_2 + \text{CO} ]
Common Reagents and Conditions:
Oxidizing Agents: Hydrogen peroxide, potassium permanganate.
Reducing Agents: Sodium borohydride.
Thermal Conditions: Heating to temperatures around 190°C for decomposition.
Major Products:
Iron(III) oxalate: Formed through oxidation.
Iron oxides: Formed through thermal decomposition.
Scientific Research Applications
Photocatalytic Applications
Iron Oxalate as a Photocatalyst
Recent studies have highlighted the use of this compound dihydrate in photocatalytic processes. For instance, this compound derived from iron ore has been effectively utilized for the degradation of organic pollutants such as rhodamine B in wastewater treatment. The synthesized this compound demonstrated superior photocatalytic activity compared to conventional methods, achieving over 85% degradation within 90 minutes. This efficiency is attributed to enhanced light absorption and improved charge separation properties of the this compound complexes .
Table: Photocatalytic Performance of this compound
Catalyst Type | Pollutant | Degradation Rate (%) | Time (min) |
---|---|---|---|
This compound | Rhodamine B | 85 | 90 |
Conventional | Rhodamine B | 74 | 90 |
Biochemical Applications
Interaction with Transferrin
This compound plays a crucial role in biochemistry, particularly concerning iron transport in the human body. It binds to transferrin, a key protein responsible for iron transport, in a manner that significantly inhibits the release of iron. This binding occurs in a symmetric bidentate fashion, making it difficult for iron to be displaced from transferrin. Such interactions suggest that elevated levels of oxalate can interfere with normal iron metabolism, potentially leading to conditions like iron deficiency anemia .
Environmental Applications
Oxidation Processes in Atmospheric Chemistry
This compound complexes are also involved in atmospheric chemistry, particularly in the oxidation of organic compounds. Studies indicate that these complexes can absorb near-UV radiation and participate in photodissociation reactions that generate reactive species capable of oxidizing dissolved organic matter. This property is essential for understanding the role of iron oxalates in tropospheric chemistry and their potential impact on environmental processes .
Industrial Applications
Recovery and Valorization
In industrial contexts, this compound is utilized for recovering oxalic acid from waste solutions generated during metal processing. The recovery process involves leaching techniques that optimize yield and allow for the reuse of by-products in further chemical processes. This approach not only enhances resource efficiency but also minimizes waste generation .
Case Study 1: Photocatalytic Degradation of Organic Pollutants
A study demonstrated the effectiveness of this compound dihydrate synthesized from natural resources in degrading rhodamine B under UV light. The results indicated that modifications to the structure of this compound could enhance its photocatalytic properties, making it a viable option for wastewater treatment.
Case Study 2: Iron Transport Interference
Research investigating the effects of high plasma oxalate levels on human transferrin revealed that substituting carbonate with oxalate significantly hampers iron release. This finding has implications for understanding nutritional deficiencies linked to autism spectrum disorders and other health conditions associated with disrupted iron metabolism.
Mechanism of Action
The mechanism of action of iron oxalate involves its ability to undergo redox reactions. In biological systems, this compound can participate in electron transfer processes, influencing iron metabolism and transport. The compound’s ability to form coordination complexes with various ligands also plays a crucial role in its chemical behavior .
Comparison with Similar Compounds
Comparison with Similar Metal Oxalates
Structural Diversity and Polymorphism
Iron oxalate exhibits structural polymorphism, a feature less common in other transition metal oxalates. Two hydrated polymorphs exist:
- α-FeC₂O₄·2H₂O: Monoclinic structure (space group P2₁/c) with lattice parameters a = 12.06 Å, b = 5.56 Å, c = 9.95 Å, and β = 128.5° .
- β-FeC₂O₄·2H₂O : Orthorhombic structure (space group Cccm) with a = 12.49 Å, b = 5.56 Å, and c = 15.46 Å .
Dehydration at 180–200°C under nitrogen produces anhydrous α- and β-FeC₂O₄. The β-polymorph adopts a monoclinic structure (space group P2₁/n) with disordered oxalate chains, while α-FeC₂O₄ remains structurally unresolved due to challenges in isolating pure phases .
In contrast, copper(II) oxalate (CuC₂O₄) forms a monoclinic structure (P2₁/n) with lattice parameters a = 5.76 Å, b = 5.07 Å, c = 5.50 Å, and β = 113.45°, featuring "zeolite-like" water adsorption rather than lattice hydration . Zinc oxalate (ZnC₂O₄) crystallizes in a tetragonal system, lacking the polymorphism seen in this compound .
Table 1: Structural Comparison of Selected Metal Oxalates
Compound | Crystal System (Hydrated) | Space Group | Lattice Parameters (Å) | Polymorphism |
---|---|---|---|---|
FeC₂O₄·2H₂O (α) | Monoclinic | P2₁/c | 12.06, 5.56, 9.95 | Yes |
FeC₂O₄·2H₂O (β) | Orthorhombic | Cccm | 12.49, 5.56, 15.46 | Yes |
CuC₂O₄·nH₂O | Monoclinic | P2₁/n | 5.76, 5.07, 5.50 | No |
ZnC₂O₄ | Tetragonal | I4/mmm | 6.23, 6.23, 5.02 | No |
Thermal Behavior and Decomposition
This compound’s thermal decomposition pathways are atmosphere-dependent:
- In air : Decomposes to α-Fe₂O₃ (hematite) at ~400°C .
- In nitrogen : Forms Fe₃O₄ (magnetite) and α-Fe via intermediate FeO (wüstite) at ~300°C .
Copper oxalate decomposes to CuO at 290°C without intermediate phases, while zinc oxalate yields ZnO at 400°C . The slower decomposition of this compound in inert atmospheres highlights its stability compared to copper and zinc analogs.
Table 2: Thermal Decomposition Products
Compound | Decomposition Temperature (°C) | Primary Products (Air) | Primary Products (Inert) |
---|---|---|---|
FeC₂O₄ | 180–400 | α-Fe₂O₃ | Fe₃O₄, α-Fe |
CuC₂O₄ | 290 | CuO | CuO |
ZnC₂O₄ | 400 | ZnO | ZnO |
Solubility and Stability
Iron(II) oxalate is poorly soluble in water (~0.02 g/100 mL), contrasting with sodium oxalate (Na₂C₂O₄), which is highly soluble (3.7 g/100 mL) . The low solubility of this compound limits its reactivity in aqueous systems but enhances its utility as a precursor for solid-state synthesis.
Biological Activity
Iron oxalate, particularly in its dihydrate form (Fe(C₂O₄)·2H₂O), is a compound that has garnered attention due to its diverse biological activities and potential applications in various fields, including medicine, environmental science, and materials science. This article delves into the biological activity of this compound, highlighting its interactions with biological systems, potential therapeutic applications, and implications in environmental contexts.
This compound can be synthesized through various methods, including co-precipitation from iron(II) sulfate solutions and oxalic acid. The resulting product is often characterized by its crystalline structure and solubility properties, which are crucial for its biological activity. The synthesis method can influence the morphology and size of the particles, affecting their reactivity and interaction with biological systems.
1. Iron Metabolism
This compound plays a significant role in iron metabolism. Studies have shown that high levels of oxalate can interfere with iron transport proteins such as transferrin (hTF). Oxalate competes with carbonate for binding sites on hTF, leading to impaired iron delivery to cells. This interaction has been particularly noted in conditions such as autism spectrum disorder (ASD), where elevated plasma oxalate levels may contribute to iron deficiency anemia by inhibiting iron release from hTF .
2. Antimicrobial Activity
Research indicates that this compound exhibits antimicrobial properties. In a study examining the effects of various iron compounds on microbial growth, it was found that this compound could inhibit the growth of specific bacterial strains. This suggests potential applications in developing antimicrobial agents or coatings for medical devices .
3. Photocatalytic Properties
This compound has demonstrated photocatalytic activity, particularly in degrading organic pollutants such as rhodamine B (RhB). In recent experiments, this compound derived from iron ore showed enhanced photocatalytic efficiency compared to conventional forms. The degradation rate exceeded 85% within 90 minutes under light irradiation, indicating its potential use in wastewater treatment applications .
Case Study 1: Autism Spectrum Disorder
A study explored the relationship between high plasma oxalate levels and iron deficiency anemia in children with ASD. The findings suggested that elevated oxalate interferes with iron transport mechanisms, leading to significant implications for managing iron deficiency in affected individuals .
Case Study 2: Waste Valorization
The valorization of industrial byproducts containing iron(II) oxalate highlights its potential as a resource recovery strategy. A study investigated the thermal conversion of waste materials containing iron(II) oxalate into valuable products like magnetite. This process not only recycles waste but also produces materials that can be used in various applications .
Research Findings
Q & A
Q. Basic: What are the optimal conditions for synthesizing iron oxalate with high crystallinity and purity?
Methodological Answer :
this compound synthesis typically involves coprecipitation or hydrothermal methods. Key parameters include:
- pH control : Maintain pH 1.5–3.0 using oxalic acid to ensure stoichiometric Fe:C₂O₄²⁻ ratios .
- Temperature : Hydrothermal synthesis at 120–180°C enhances crystallinity .
- Characterization : Use XRD to confirm phase purity (e.g., FeC₂O₄·2H₂O vs. anhydrous forms) and FTIR to verify oxalate ligand coordination (e.g., ν(C=O) at 1,630 cm⁻¹) .
Q. Advanced: How can computational methods resolve contradictions in reported catalytic activity of this compound in Fenton-like reactions?
Methodological Answer :
Discrepancies often arise from variations in surface defects or Fe²⁺/Fe³⁺ ratios. Address this via:
- Density Functional Theory (DFT) : Model oxygen vacancy impacts on •OH generation .
- In situ spectroscopy : Track Fe oxidation states during H₂O₂ activation using XANES .
- Controlled synthesis : Compare defect-engineered vs. pristine samples to isolate activity drivers .
Q. Basic: What analytical techniques are critical for assessing this compound’s thermal decomposition kinetics?
Methodological Answer :
- Thermogravimetric Analysis (TGA) : Identify decomposition stages (e.g., FeC₂O₄ → Fe₃O₄ + CO₂ at 250–400°C) .
- Kinetic modeling : Apply Flynn-Wall-Ozawa method to calculate activation energy from TGA data .
- Gas chromatography (GC) : Quantify CO/CO₂ release to validate decomposition pathways .
Q. Advanced: How to reconcile conflicting reports on this compound’s photoreactivity under visible light?
Methodological Answer :
Contradictions may stem from differences in light sources or charge recombination rates. Mitigate via:
- Standardized testing : Use AM 1.5G solar simulators with calibrated irradiance .
- Transient absorption spectroscopy : Measure carrier lifetimes to correlate with photocatalytic efficiency .
- Doping studies : Introduce Ti⁴+ or Mn²+ to alter bandgap and assess reproducibility .
Q. Basic: What role does pH play in this compound polymorph formation?
Methodological Answer :
- Low pH (1.5–2.5) : Favors FeC₂O₄·2H₂O due to suppressed Fe³⁺ hydrolysis .
- Neutral pH : Promotes amorphous phases or mixed oxyhydroxide-oxalate species .
- Experimental design : Use in situ pH monitoring and SEM to track morphology evolution .
Q. Advanced: What mechanistic insights explain this compound’s role in selective organic oxidation?
Methodological Answer :
- Radical trapping : Use ethanol or benzoquinone to identify •OH vs. O₂•− contributions .
- Isotopic labeling : ¹⁸O₂ tracing to distinguish lattice oxygen vs. atmospheric O₂ involvement .
- Operando Raman : Monitor Fe-O bonding changes during catalysis .
Q. Basic: How to ensure reproducibility in this compound synthesis across laboratories?
Methodological Answer :
- Protocol standardization : Report exact molar ratios, aging times, and drying conditions .
- Inter-lab validation : Share samples for XRD/FTIR cross-testing to identify batch variability .
- Purity thresholds : ICP-MS for trace metal quantification (e.g., Al³⁺, Cu²⁺ contaminants) .
Q. Advanced: Can this compound nanoparticles mitigate environmental risks of legacy heavy metals?
Methodological Answer :
- Structure-activity studies : Correlate nanoparticle size (via TEM) with Pb²⁺/Cd²⁺ adsorption efficiency .
- Column leaching tests : Simulate soil conditions to assess long-term stability of metal-oxalate complexes .
- Ecotoxicity assays : Use Daphnia magna or Vibrio fischeri to evaluate environmental impact .
Properties
CAS No. |
2944-66-3 |
---|---|
Molecular Formula |
C6Fe2O12 |
Molecular Weight |
375.75 g/mol |
IUPAC Name |
iron(3+);oxalate |
InChI |
InChI=1S/3C2H2O4.2Fe/c3*3-1(4)2(5)6;;/h3*(H,3,4)(H,5,6);;/q;;;2*+3/p-6 |
InChI Key |
VEPSWGHMGZQCIN-UHFFFAOYSA-H |
SMILES |
C(=O)(C(=O)[O-])[O-].C(=O)(C(=O)[O-])[O-].C(=O)(C(=O)[O-])[O-].[Fe+3].[Fe+3] |
Canonical SMILES |
C(=O)(C(=O)[O-])[O-].C(=O)(C(=O)[O-])[O-].C(=O)(C(=O)[O-])[O-].[Fe+3].[Fe+3] |
Key on ui other cas no. |
15843-42-2 446884-61-3 2944-66-3 |
Pictograms |
Irritant |
Synonyms |
Acid, Oxalic Aluminum Oxalate Ammonium Oxalate Chromium (2+) Oxalate Chromium (3+) Oxalate (3:2) Chromium Oxalate Diammonium Oxalate Dilithium Oxalate Dipotassium Oxalate Disodium Oxalate Ferric Oxalate Iron (2+) Oxalate (1:1) Iron (3+) Oxalate Iron Oxalate Magnesium Oxalate Magnesium Oxalate (1:1) Manganese (2+) Oxalate (1:1) Monoammonium Oxalate Monohydrogen Monopotassium Oxalate Monopotassium Oxalate Monosodium Oxalate Oxalate, Aluminum Oxalate, Chromium Oxalate, Diammonium Oxalate, Dilithium Oxalate, Dipotassium Oxalate, Disodium Oxalate, Ferric Oxalate, Iron Oxalate, Magnesium Oxalate, Monoammonium Oxalate, Monohydrogen Monopotassium Oxalate, Monopotassium Oxalate, Monosodium Oxalate, Potassium Oxalate, Potassium Chromium Oxalate, Sodium Oxalic Acid Potassium Chromium Oxalate Potassium Oxalate Potassium Oxalate (2:1) Sodium Oxalate |
Origin of Product |
United States |
Retrosynthesis Analysis
AI-Powered Synthesis Planning: Our tool employs the Template_relevance Pistachio, Template_relevance Bkms_metabolic, Template_relevance Pistachio_ringbreaker, Template_relevance Reaxys, Template_relevance Reaxys_biocatalysis model, leveraging a vast database of chemical reactions to predict feasible synthetic routes.
One-Step Synthesis Focus: Specifically designed for one-step synthesis, it provides concise and direct routes for your target compounds, streamlining the synthesis process.
Accurate Predictions: Utilizing the extensive PISTACHIO, BKMS_METABOLIC, PISTACHIO_RINGBREAKER, REAXYS, REAXYS_BIOCATALYSIS database, our tool offers high-accuracy predictions, reflecting the latest in chemical research and data.
Strategy Settings
Precursor scoring | Relevance Heuristic |
---|---|
Min. plausibility | 0.01 |
Model | Template_relevance |
Template Set | Pistachio/Bkms_metabolic/Pistachio_ringbreaker/Reaxys/Reaxys_biocatalysis |
Top-N result to add to graph | 6 |
Feasible Synthetic Routes
Disclaimer and Information on In-Vitro Research Products
Please be aware that all articles and product information presented on BenchChem are intended solely for informational purposes. The products available for purchase on BenchChem are specifically designed for in-vitro studies, which are conducted outside of living organisms. In-vitro studies, derived from the Latin term "in glass," involve experiments performed in controlled laboratory settings using cells or tissues. It is important to note that these products are not categorized as medicines or drugs, and they have not received approval from the FDA for the prevention, treatment, or cure of any medical condition, ailment, or disease. We must emphasize that any form of bodily introduction of these products into humans or animals is strictly prohibited by law. It is essential to adhere to these guidelines to ensure compliance with legal and ethical standards in research and experimentation.